Abstract
Highly active olefin metathesis catalysts were prepared by thermal spreading MoO3 and/or MoO2(acac)2 on MWW zeolites (MCM-22, delaminated MCM-56) and on two-dimensional MFI (all in NH4+ form). The catalysts‘ activities were tested in the metathesis of neat 1-octene (as an example of a longer chain olefin) at 40 °C. Catalysts with 6 wt % or 5 wt % of Mo were used. The acidic character of the supports had an important effect on both the catalyst activity and selectivity. The catalyst activity increases in the order 6MoO3/HZSM-5(25) (Si/Al = 25) << 6MoO2(acac)2/MCM-22(70) < 6MoO3/2D-MFI(26) < 6MoO3/MCM-56(13) < 6MoO3/MCM-22(28) reflecting both the enhancing effect of the supports‘ acidity and accessibility of the catalytic species on the surface. On the other hand the supports‘ acidity decreases the selectivity to the main metathesis product C14 due to an acid-catalyzed double bond isomerization (followed by cross metathesis) and oligomerization. 6MoO3/2D-MFI(26) with a lower concentration of the acidic centres resulting in catalysts of moderate activity but with the highest selectivity.
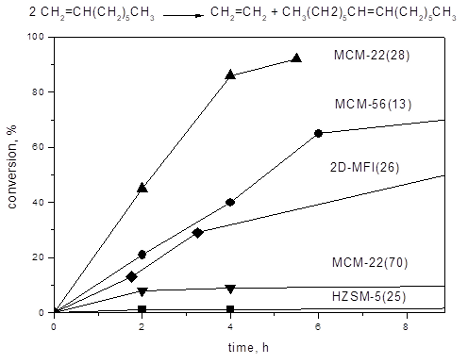
Graphical Abstract
Introduction
Molybdenum oxide on silica, alumina or silica-alumina belongs to the well-known and long-time used metathesis catalysts [1]. Albeit typical ill-defined catalysts they are still popular as relatively cheap catalysts finding industrial applications especially in the treatment of low olefins [2-5]. Their catalytic activity depends on many factors, especially on Mo loading, support acidity, and pre-reaction activations. Surface isolated MoO4 tetrahedra were proved as the main precursors of the catalytic species [6,7], thus the perfect dispersion of MoO3 on the surface is a crucial precondition for a high catalytic activity. The mechanisms of transformation of these precursors to the surface Mo carbenes as real catalytically active species has been suggested [6,7]. The replacement of ordinary silicas for mesoporous molecular sieves SBA-15 or MCM-41 increased the catalyst activity substantially, which allowed performing the metathesis of long chain olefins under mild reaction conditions [8-10]. The positive effect of these supports on the catalyst activity was ascribed to their high surface areas enhancing the spreading of MoO3 molecules on the surface and large pores increasing the substrates/products transport rate.
Microporous zeolites like HZSM-5 impregnated by ammonium heptamolybdate solutions were used for the metathesis of low olefins (ethylene, propylene, butenes) [11-13]. In the case of bulkier substrates they suffer, however, of micropore size limitations. To overcome these limitations a decrease in crystal size and the application of two-dimensional zeolites can be used [14-17]. Two dimensional 2D-MFI and MWW delaminated zeolite MCM-56, which have been prepared recently [18-21], represent two types of these materials, which exhibit relatively high surface areas and high accessibility of catalytic sites on the surface as well [22]. Therefore, we supported MoO3 and/or MoO2(acac)2 on (i) 2D-MFI (and ordinary HZSM-5 for comparison) and similarly on (ii) MCM-56 and its 3D analogue MCM-22 (both in NH4+ form) and examined their activity in the metathesis of neat 1-octene (Scheme 1) under ambient pressure and 40 °C. According to our best knowledge, none of these materials have been tested as supports for MoO3 based catalysts for metathesis of higher alkenes up to now. MoOx on MCM-22 combined with γ-Al2O3 was used in cross metathesis of 2-butene and ethylene in a stream (125 °C, 1 MPa) [23]. MCM-22, and MCM-56 were also used as supports for Hoveyda–Grubbs type hybrid catalysts active in metathesis of long-chain unsaturated esters [24].
Results and Discussion
Catalyst preparation and characterization
XRD patterns and texture properties (Table 1, Figure 1 A,B,C,D) of prepared MCM-22, MCM-56 and 2D-MFI zeolites proved a high quality of these supports. For catalyst labelling following the mode has been adopted: x MoO3/MCM-22(y), where x = Mo concentration in wt % Mo, y = Si/Al molar ratio. After spreading Mo compounds over the support surface areas (SBET, Sext) as well as void volumes (V) decreased. Similar reduction of these quantities has been already observed earlier [9,10,24]. For x MoO3/MCM-22(28), XRD patterns of catalysts are similar to those of their parents approximately up to x = 6 wt % of Mo (0.9 Mo atoms per nm2). At higher Mo concentrations signals of crystalline MoO3 appeared (marked with * in Figure 1 A,B,D). It suggests 6 wt % of Mo being the optimal Mo loading. On the other hand, x MoO3/MCM-22(70) catalyst with x = 6 wt % Mo exhibited slight MoO3 signals, when prepared from MoO3 probably due to the lower surface area (especially external one) comparing with MCM-22(28). However, when MoO2(acac)2 was used as a source of Mo, catalysts with 6 wt % (and lower) content of Mo did not exhibit any MoO3 signals. It is consistent with the previous observation that MoO2(acac)2 provided better catalyst than MoO3 [9]. XRD patterns of 6MoO3/MCM-56(13) and 6MoO3/2D-MFI(26) indicated also a good MoO3 spreading, contrary to 6MoO3/HZSM-5(25) where MoO3 signals were clearly visible, probably as a result of lower external surface area.
Table 1: Texture properties of catalysts and corresponding supports.
catalyst |
SBET
(m2/g) |
Sext
(m2/g) |
V
(cm3/g) |
|
---|---|---|---|---|
1 | MCM-22(28) | 455 | 119 | 0.59 |
2 | 6MoO3/MCM-22(28) | 423 | 119 | 0.38 |
3 | 6MoO2(acac)2/MCM-22(28) | 426 | 94 | 0.57 |
4 | MCM-22(70) | 421 | 58 | 0.29 |
5 | 6MoO3/MCM-22(70) | 180 | 39 | 0.25 |
6 | 6MoO2(acac)2/MCM-22(70) | 355 | 41 | 0.24 |
7 | 2D-MFI(26) | 565 | 343 | 0.61 |
8 | 6MoO3/2D-MFI(26) | 478 | 221 | 0.57 |
9 | MCM-56(13) | 469 | 164 | 0.57 |
10 | 6MoO3/MCM-56(13) | 269 | 129 | 0.55 |
11 | HZSM-5(25) | 410 | 44 | 0.23 |
12 | 6MoO3/HZSM-5(25) | 388 | 38 | 0.23 |
SBET = BET area, V = total void volume (p/p0 = 0.95), Sext = external surface (from t-plot).
![[1860-5397-14-272-1]](/bjoc/content/figures/1860-5397-14-272-1.png?scale=2.0&max-width=1024&background=FFFFFF)
Figure 1: A,B,C,D: XRD patterns of parent supports and catalysts used. Asterisk marks MoO3.
Figure 1: A,B,C,D: XRD patterns of parent supports and catalysts used. Asterisk marks MoO3.
Contrary to the all-siliceous mesoporous sieves (like SBA-15) which are neutral, zeolites are acidic and their acidity (both Brønsted and Lewis-type) plays an important role for catalysis. The acid site concentrations of zeolitic supports and the corresponding catalysts measured using FTIR spectroscopy of adsorbed pyridine are shown in Table 2, while the relevant IR spectra are shown in Supporting Information File 1 (Figures S1–S5). It is seen that all supports contained both Brønsted and Lewis acid sites of various strength. MCM-22(28) and MCM-56(13) exhibited the highest concentrations of acid sites (both Brønsted and Lewis) in accord with their highest Al concentrations. The acid sites concentrations of MCM-22(70) and 2D-MFI(26) were lower and close to each other. The Brønsted acid site concentration of HZSM-5(25) was as high as that of MCM-22(28), however, its Lewis acid site concentration was significantly lower. After supporting Mo compounds the concentrations of Brønsted acid sites decreased significantly which may indicate that MoOx species reacted predominantly with Brønsted acid sites of the supports. It is manifested by intensity decrease of the band in the region 3609–3625 cm−1, ascribed to OH vibration in the Si–O(H)–Al acid site (see Supporting Information File 1, Figures S1–S5) [25]. On the other hand, the concentrations of Lewis acid sites in the catalysts was slightly higher compared to the parent supports. It may be explained by the formation of some amount of Mo in a lower oxidation state which has been already described for siliceous supports (MCM-41, SBA-15) [9,26].
Table 2: Acid site concentrations in catalysts and corresponding supports.a
sample | c(B)b, mmol/g | c(L)c, mmol/g | ||||||
---|---|---|---|---|---|---|---|---|
150 °C | 250 °C | 350 °C | 450 °C | 150 °C | 250 °C | 350 °C | 450 °C | |
MCM-22(70) | 0.11 | 0.12 | 0.09 | 0.02 | 0.06 | 0.04 | 0.03 | 0.03 |
6MoO3/MCM-22(70) | 0.02 | 0.01 | – | – | 0.10 | 0.01 | 0.01 | – |
6MoO2(acac)2/MCM-22(70) | 0.06 | 0.06 | 0.05 | 0.02 | 0.16 | 0.07 | 0.04 | 0.03 |
MCM-22(28) | 0.29 | 0.32 | 0.30 | 0.19 | 0.13 | 0.09 | 0.08 | 0.06 |
6MoO3/MCM-22(28) | 0.07 | 0.09 | 0.07 | 0.04 | 0.31 | 0.10 | 0.03 | 0.01 |
6MoO2(acac)2/ MCM-22(28) | 0.09 | 0.07 | 0.05 | 0.02 | 0.23 | 0.08 | 0.03 | 0.01 |
2D-MFI(26) | 0.13 | 0.13 | 0.08 | 0.02 | 0.07 | 0.07 | 0.06 | 0.04 |
6MoO3/2D-MFI(26) | 0.06 | 0.04 | 0.03 | 0.01 | 0.10 | 0.03 | 0.01 | – |
HZSM-5(25) | 0.29 | 0.28 | 0.22 | 0.07 | 0.04 | 0.03 | 0.02 | 0.02 |
6MoO3/HZSM-5(25) | 0.14 | 0.12 | 0.09 | 0.03 | 0.17 | 0.07 | 0.04 | 0.04 |
MCM-56(13) | 0.23 | 0.17 | 0.13 | 0.04 | 0.18 | 0.12 | 0.09 | 0.08 |
MoO3/MCM-56(13) | 0.04 | 0.03 | 0.01 | – | 0.17 | 0.06 | 0.03 | 0.02 |
aDetermined by FTIR. bBrønsted acid site. cLewis acid site.
Catalytic activity
MCM-22-based catalysts
Na+ forms of zeolites turned out to be unsuitable supports for metathesis catalysts. For example, by supporting MoO3 on MCM-22(28) in Na+ form (6 wt % of Mo) we obtained material providing only 0.5% 1-octene conversion in 19 h (1-octene/Mo = 320, t = 40 °C). Therefore, we converted Na+ forms to NH4+ forms, which were used for supporting Mo compounds by thermal spreading method.
The time development of 1-octene conversion over 6MoO3/MCM-22(28) is shown in Figure 2. The GC chromatogram of the final product is shown in Figure S6 (in Supporting Information File 1). It is seen that in addition to the main metathesis product (7-tetradecene), alkenes from C13 to C9 are present in considerable amounts. It is a consequence of the 1-octene double bond isomerization followed by cross metathesis. Moreover, a certain amount of oligomers (mainly dimers) were also observed in the reaction mixtures. Both isomerization and oligomerization are due to the acidic character of the support (vide infra). In addition to the total conversion of 1-octene (Ktot), the conversion to all metathesis products (Kmet), and the conversion to tetradecene (KC14) calculated according to the following equations are plotted in Figure 2.
where mi and Mi (i = 9–14) are weight amounts and molecular weights of alkenes from C9 to C14; md, mt and Md, Mt are weight amounts and molecular weights of octene dimers and trimers, respectively; mC8 is weight amount of octene (all isomers) and MC8 is the molecular weight of octene.
![[1860-5397-14-272-2]](/bjoc/content/figures/1860-5397-14-272-2.png?scale=2.0&max-width=1024&background=FFFFFF)
Figure 2: Conversion vs time curves for 1-octene metathesis over 6MoO3/MCM-22(28) and 6MoO3/SBA-15. Neat 1-octene, 1-octene/Mo = 320, t = 40 °C.
Figure 2: Conversion vs time curves for 1-octene metathesis over 6MoO3/MCM-22(28) and 6MoO3/SBA-15. Neat 1-oc...
For comparison, the conversion curve over 6MoO3/SBA-15 is added in Figure 2. 6MoO3/SBA-15 was prepared from all-siliceous SBA-15 (SBET = 877m2/g, V = 1.07cm3/g, pore diameter D = 6.4 nm) and it is known as a very active and selective catalyst [9,10]. Under reaction conditions applied the selectivity to tetradecene was about 98% during the whole experiment, and therefore only Ktot is plotted in Figure 2 in this case.
Both Ktot and Kmet for 6MoO3/MCM-22(28) were significantly higher than the total conversion for 6MoO3/SBA-15 (Figure 2). Conversions to oligomers (Kol = Ktot − Kmet) were about 12% (at 2 h) and practically did not change in the further course of the reaction. However, the conversions to tetradecene were rather low (maximum conversion about 20% was achieved). Higher catalytic activity of molybdenum oxide on zeolitic support in metathesis may be ascribed to the higher acidity of supports. The enhancing effect of Brønsted acidity on the catalytic activity has been already described [6] and it assumed that most of Mo active species in zeolite-based catalysts are formed by reacting molybdenum oxide with Si-O(H)-Al groups [12,27]. Similarly, Lim et al. showed recently [28], that Brønsted acid sites improve dispersion of molybdenum oxide on the surface. Moreover, for related system based on tungsten oxide in zeolite, it was suggested using high resolution STEM that Brønsted acid sites in proximity to metathesis active sites facilitate olefin adsorption and metallocycle formation [29]. Such mechanism may be effective also for Mo catalysts. The decrease in the selectivity due to isomerization and/or oligomerization seems to be an unavoidable cost for this activity enhancement.
It is known for molybdenum oxide catalysts, that with increasing Mo loading the catalytic activities increase up to maximum value [6,10]. At higher loadings the molybdenum oxide spreading on the surface became imperfect and catalytically inactive bulk MoO3 appears. The effect of increasing Mo loading on catalyst activity for MCM-22(28)-based catalyst is shown in Table 3.
Table 3: The effect of Mo loading on catalyst activity in 1-octene metathesis.a
catalyst | reaction time, h | Ktot, % | Kmet, % | Kol, % | KC14, % |
---|---|---|---|---|---|
6MoO3/MCM-22(28) |
2
4 6 |
45
86 92 |
33
75 79 |
12
11 13 |
12
20 20 |
8MoO3/MCM-22(28) |
2
4 6.5 22 |
21
41 58 85 |
15
35 51 77 |
6
6 7 8 |
10
18 25 36 |
10MoO3/MCM-22(28) |
2
4 6 |
2.6
3.4 4 |
0.6
0.7 1 |
2
2.7 3 |
0.6
0.7 1 |
a50 mg Catalyst, 1.5 mL 1-octene, 40 °C.
For 8MoO3/MCM-22(28) XRD pattern shows a small amount of bulk MoO3 (marked with asterisk in Figure 1A). In accord with this, the conversions fell down in comparison with 6MoO3/MCM-22(28), the selectivity, however, slightly increased: the amount of oligomers was reduced and the selectivity to the tetradecene approximately doubled. It suggests that more acid sites were covered by MoOx species and oligomerization and isomerization ability of catalysts decreased. However, further increase in the Mo loading to 10 wt % in 10MoO3/MCM-22(28) led nearly to the lost of catalytic activity, which is explained by deposition of Mo in the catalytically inactive bulk MoO3. Correspondingly, very intensive diffraction lines of the bulk MoO3 appeared in the XRD pattern of 10MoO3/MCM-22(28) (see Figure 1A).
To reduce isomerization and oligomerization ability of MCM-22-based catalysts we prepared zeolite with Si/Al = 70 (and therefore with lower acidity – vide supra): MCM-22(70). The results showing the catalytic behavior of the prepared MCM-22(70)-based catalysts 6MoO2(acac)2/MCM-22(70), 5MoO2(acac)2/MCM-22(70), and 4MoO2(acac)2/MCM-22(70) are collected in Table 4.
Table 4: 1-Octene metathesis over MCM-22(70)-based catalysts.a
catalyst | reaction time, h | Ktot, % | Kmet, % | Kol, % | KC14, % |
---|---|---|---|---|---|
6MoO3/MCM-22(70) |
2
4.5 6 |
2
3 2 |
– | – | – |
6MoO2(acac)2/MCM-22(70) |
2
4 22 |
8
9 11.5 |
7.5
8 10.5 |
0.5
1 1 |
5
6 7 |
5MoO2(acac)2/MCM-22(70) |
2.3
3.3 20 |
8
11 35 |
7
10 32 |
1
1 3 |
5
7 17 |
4MoO2(acac)2/MCM-22(70) |
2
4 21 |
11
16 16 |
11
15 15 |
0
1 1 |
10
14 14 |
a50 mg Catalyst, 1.5 mL 1-octene, 40 °C.
XRD pattern of 6MoO3/MCM-22(70) exhibited some amount of bulk MoO3 (Figure 1B). Evidently on this less acidic support the MoO3 spreading is not perfect, which explains its negligible activity in metathesis reaction. However, using bis(acetylacetonate) complex MoO2(acac)2 as a source of Mo we obtained 6MoO2(acac)2/MCM-22(70), 5MoO2(acac)2/MCM-22(70), and 4MoO2(acac)2/MCM-22(70) exhibiting no signals of bulk MoO3 in XRD pattern (Figure 1B) and showing a mild metathesis activity. The highest conversion Ktot = 35% (after 20 h) was achieved over 5MoO2(acac)2/MCM-22(70). Oligomerization activity of all these catalysts was considerably lower in comparison with that of 6MoO3/MCM-22(28) (Kol = 1% only). However, the isomerization was not suppressed and conversion to tetradecene KC14 was low.
MCM-56-based catalysts
Conversion curves for the 1-octene metathesis over 6MoO3/MCM-56(13) under standard conditions are displayed in Figure 3. In spite of the 2D character of support the conversions over 6MoO3/MCM-56(13) were significantly lower in comparison with 6MoO3/MCM-22(28): the initial reaction rate (calculated at reaction time = 2 h) being about a half of the initial reaction rate over 6MoO3/MCM-22(28). On the other hand the extent of oligomerization was practically the same (for final product the oligomerization selectivity was 14%) and the extent of cross metathesis was even higher (the selectivity to tetradecene was only 15%). The crystals of MCM-22 (see SEM image in Supporting Information File 1, Figure S7) consist of very thin platelets and therefore a great amounts of 12-membered ring cups of MWW structure are on crystal exterior [18]. These cups as we assume host MoOx species. Although MCM-56(13) as 2D zeolite consists of very thin layers, these layers may be curled and packed, which prevents the access of substrate molecules to the most of 12MR cups (for MCM-56(13) morphology see Supporting Information File 1, Figure S8). This may explain the lower activity of 6MoO3/MCM-56(13) compared with 6MoO3/MCM-22(28). Similarly, a higher activity of MCM-22 in comparison with MCM-56 has been observed in toluene disproportionation [18] and also for RCM of citronellene over immobilized Ru catalysts the activity of catalyst based on MCM-56 was not higher than that based on MCM-22 [24].
![[1860-5397-14-272-3]](/bjoc/content/figures/1860-5397-14-272-3.png?scale=2.0&max-width=1024&background=FFFFFF)
Figure 3: Conversion vs time curves for the 1-octene metathesis over 6MoO3/MCM-56(13). Neat 1-octene, 1-octene/Mo = 320, t = 40 °C.
Figure 3: Conversion vs time curves for the 1-octene metathesis over 6MoO3/MCM-56(13). Neat 1-octene, 1-octen...
MFI-based catalysts
The comparison of conversion curves for 1-octene over 6MoO3/2D-MFI(26) and 6MoO3/HZSM-5(25) under standard conditions is given in Figure 4. It is seen that 6MoO3/HZSM-5(25) exhibited only negligible activity (Ktot = Kmet = 3% after 20 h) in accord with poor MoO3 spreading (see Figure 1D). Despite the high acidity of the support, a poor accessibility of relevant surface OH groups during the thermal spreading process and a poor accessibility of possible active sites by substrate molecule during metathesis may cause 6MoO3/HZSM-5(25) to be practically inactive. On the other hand, over 6MoO3/2D-MFI(26) about 90% conversion was achieved for the same reaction time (20 h). The initial reaction rate over 6MoO3/2D-MFI(26) was only slightly lower than that over 6MoO3/MCM-56(13) and about one half of that over 6MoO3/MCM-22(28). Contrary to 6MoO3/MCM-22(28) the oligomerization activity of 6MoO3/2D-MFI(26) was reduced (Kol was from 1% to 5%) and the selectivity to tetradecene was higher (for final conversions KC14/Kmet = 0.41 and 0.25 for 6MoO3/2D-MFI(28) and 6MoO3/MCM-22(28), respectively). Lower acidity of 6MoO3/2D-MFI(28) may explain the lower extent of oligomerization and isomerization reactions and increased tetradecene selectivity. Lower acidity may also bring about the reduced activity as compared with 6MoO3/MCM-22(28); however, different structures of MCM-22 and MFI do not allow simple comparison.
![[1860-5397-14-272-4]](/bjoc/content/figures/1860-5397-14-272-4.png?scale=2.0&max-width=1024&background=FFFFFF)
Figure 4: Conversion vs time curves for 1-octene metathesis over 6MoO3/2D-MFI(26) and 6MoO3/HZSM-5(25). Neat 1-octene, 1-octene/Mo = 320, t = 40 °C.
Figure 4: Conversion vs time curves for 1-octene metathesis over 6MoO3/2D-MFI(26) and 6MoO3/HZSM-5(25). Neat ...
The accompanying oligomerization activity
The experiments with Mo-free zeolites (Figure 5a,b) confirmed that the oligomerization activity was connected with the support itself. In these “blank” experiments the reaction conditions, as well as pretreatment mode were the same as for Mo oxide catalysts. No metathesis products were observed, only 1-octene oligomerization and double bond isomerization occurred. Figure 5a shows 1-octene oligomerization over MCM-22(28) and MCM-22(70). Families of dimers and trimers (in weight ratio dimers/trimers approximately 20:1 for the final conversions) were detected, isolation and characterization of individual dimers/trimer was not possible. It was visible from GC, that isomerization of starting 1-octene also occurred, however, the exact quantification was not possible. The oligomerization rate was higher for MCM-22(28) in accord with its higher acidity as compared with MCM-22(70). The extent of oligomerization in these blank experiments is several times higher than that achieved over metathesis catalysts: it may be due to the partial capping of support acid sites with Mo species catalysts and also due the parallel consumption of 1-octene in metathesis.
![[1860-5397-14-272-5]](/bjoc/content/figures/1860-5397-14-272-5.png?scale=2.0&max-width=1024&background=FFFFFF)
Figure 5: Conversion to oligomers for 1-octene over MCM-22(28) and MCM-22(70) (a) and conversion to oligomers for 1-octene, 2-octene, 3-octene, and 4-octene over MCM-22(28) (b). 50 mg Support, 1.5 mL octene, 40 °C.
Figure 5: Conversion to oligomers for 1-octene over MCM-22(28) and MCM-22(70) (a) and conversion to oligomers...
Figure 5b shows oligomerization of 1-octene, 2-octene (cis + trans), 3-octene (trans), and 4-octene (trans) over MCM-22(28). It is seen that the initial reaction rate decreases in the order 1-octene ≈ 2-octene > 3-octene > 4-octene. The low-temperature oligomerization of alkenes over zeolite was studied as concerns industrially important low alkenes oligomerization and lower reactivity of internal alkenes in comparison with 1-alkenes was also recognized [30,31]. The reduced activity of 3- and 4-octenes in oligomerization might explain the fact, that in our metathesis experiments the accompanying oligomerization occurred practically only in the beginning of the reaction. In later stages when most of 1-octene was isomerized to 3- and 4-octenes only little increase in oligomer amounts was observed.
Conclusion
3D and 2D zeolites of MWW (MCM-22 and MCM-56) and MFI topologies were used for the first time as supports for the preparation of highly active molybdenum oxide metathesis catalysts. The catalysts, prepared by thermal spreading of MoO3 and/or MoO2(acac)2 on these supports in NH4+ forms (6 wt % and/or 5 wt % of Mo) were tested in neat 1-octene metathesis under mild conditions (batch reactor, atmospheric pressure, 40 °C).
The catalyst activity (expressed as Ktot values at the reaction time = 2 h) decreased in the order 6MoO3/MCM-22(28) > 6MoO3/MCM-56(13) > 6MoO3/2D-MFI(26) > 6MoO2(acac)2/MCM-22(70) >> 6MoO3/HZSM-5(25). This activity order reflects two effects enhancing the activity: (i) support acidity and (ii) structure characteristics ensuring good accessibility of active species by substrate molecules. The most active 6MoO3/MCM-22(28) exhibited a significantly higher activity than that of a similar catalyst supported on siliceous mesoporous molecular sieve SBA-15.
Due to the catalyst acidity accompanying reactions occurred: (i) 1-octene double bond isomerization followed by cross metathesis and (ii) 1-octene oligomerization (mainly dimerization). The extent of these reactions depends strongly on the support acidity. Highly acidic supports MCM-22(28) and MCM-56(13) delivered a catalyst of rather low selectivity (up to 14% conversion to oligomers, 15–20% conversion to tetradecene at about Ktot = 90%). Less acidic supports – MCM-22(70) and 2D-MFI(26) gave rise catalysts of significantly higher selectivity: conversion to oligomers was reduced to 1%, double bond isomerization and cross metathesis proceeded in less extent, so selectivity to tetradecene increased (e.g., for 2D-MFI(26) to 35% at Ktot = 90%).
It is seen that for the metathesis of longer chain hydrocarbons like 1-octene, supports ensuring a good access of bulkier substrate to the active centers are necessary. The acidity of the support increases the catalyst activity, however, simultaneously with decrease of the catalyst selectivity. 2D-MFI(26) due to its moderate acidity and 2D character results in catalysts of moderate activity but of the highest selectivity.
With the described catalysts 1-octene was converted into a mixture of higher olefins: in addition to tetradecene as a homometathesis product, olefins of 9–13 C atoms from cross metathesis and C16 dimers were formed in various extent. Therefore, the described catalysts may find application especially if a mixture of higher olefins is desired, for example in the preparation of detergents, lubricants etc.
Experimental
Catalyst preparation and characterization
The zeolite supports MCM-22 and MCM-56 were prepared according to [32,33], 2D-MFI was synthesized according to [21]. HZSM-5 (CBV 5524) was purchased from Zeolyst. Na+ forms of zeolites were converted to NH4+ form by three-fold treatment with 1.0 M NH4NO3 solution at room temperature for 3 h. The supports were characterized by XRD (Bruker AXS D8 Advance diffractometer with a graphite monochromator and a Vantec-1 position sensitive detector using Cu Kα radiation in Bragg−Brentano geometry) and by N2 adsorption (77 K, Micromeritics GEMINI II 2370 volumetric Surface Area Analyzer). Molybdenum(VI) oxide (Sigma-Aldrich) and bis(acetylacetonato)dioxomolybdenum(VI) (Aldrich) as sources of Mo oxide species were used for catalyst preparation using the thermal spreading method (500 °C, 8 h). SEM images were recorded using a JEOL JSM-5500LV microscope.
The concentrations of Lewis (cL) and Brønsted (cB) acid sites were determined by FTIR spectroscopy of adsorbed pyridine (Py) using a Nicolet 6700 with a transmission MCT/B detector. The zeolites were pressed into self-supporting wafers with a density of 8.0–12 mg·cm–2 and activated in situ at T = 450 °C and p = 5·10–5 torr for 4 h. Pyridine adsorption was carried out at 150 °C and a partial pressure of 3.5 torr for 20 min followed by desorption for 20 min at 150, 250, 350 or 450 °C. Before adsorption, pyridine was degassed by freeze–pump–thaw cycles. All spectra were recorded with a resolution of 4 cm–1 by collecting 128 scans for a single spectrum at room temperature. The spectra were recalculated using a wafer density of 10 mg·cm–2. cL and cB were evaluated from the integral intensities of bands at 1454 cm–1 (cL) and 1545 cm–1 (cB) using extinction coefficients, ε(L)=2.22 cm·mmol–1 and ε (B)=1.67 cm·mmol–1[34].
For elemental analysis ICP OES (iCAP 7000, Thermo Scientific) was used. About 50 mg of the catalyst was digested in a mixture of HF, HCl, and HNO3 (1:2:2). The samples were placed in a Berghof microwave in a closed vessel at T = 140 °C for 35 min. Saturated solution of H3BO3 was then added for complexation of the excess of HF. After digestion solutions under analysis were collected in 250 mL flasks and diluted with ultra pure water.
Catalytic experiments
Catalytic experiments were carried out in an argon atmosphere using a vacuum argon line. 1-Octene (Aldrich, 98%) was passed through alumina and stored with Na. The content of water in 1-octene was about 5 ppm. 2-Octene (Alfa-Aesar, 98%), trans-3-octene (Alfa-Aesar, 97%) and trans-4-octene (Aldrich) were purified in a similar way. In a typical experiment 50 mg of catalyst (6 wt % of Mo) was used. Before reaction catalyst was pretreated in vacuo at 500 °C for 30 min. After cooling to 40 °C, the reactor was filled with Ar and neat 1-octene (1-octene/Mo ratio = 320) was added under stirring. The reaction progress was followed by GC analysis of reaction mixture samples taken at given intervals. Individual compounds were identified by GC/MS. A high-resolution gas chromatograph Agilent 6890 with a DB-5 column (length: 50 m, inner diameter: 320 μm, stationary phase thickness: 1 μm), equipped with a 7683 Automatic Liquid Sampler and a FID detector and GC/MS (ThermoFinnigan, FOCUS DSQ II single Quadrupole) were used. Conversions were calculated from the mass balance.
Supporting Information
Supporting Information File 1: IR spectra of catalysts, GC of reaction products, and SEM images of catalysts. | ||
Format: PDF | Size: 527.0 KB | Download |
References
-
Ivin, K. J.; Mol, J. C. Applications of the Olefin Metathesis Reaction. Olefin Metathesis and Metathesis Polymerization, 2nd ed.; Academic Press: London, 1997; pp 397–410. doi:10.1016/b978-012377045-5/50018-5
Return to citation in text: [1] -
Hahn, T.; Bentrup, U.; Armbrüster, M.; Kondratenko, E. V.; Linke, D. ChemCatChem 2014, 6, 1664–1672. doi:10.1002/cctc.201400040
Return to citation in text: [1] -
Zhang, D.; Li, X.; Liu, S.; Huang, S.; Zhu, X.; Chen, F.; Xie, S.; Xu, L. Appl. Catal., A 2012, 439-440, 171–178. doi:10.1016/j.apcata.2012.07.002
Return to citation in text: [1] -
Hahn, T.; Kondratenko, E. V.; Linke, D. Chem. Commun. 2014, 50, 9060–9063. doi:10.1039/c4cc01827c
Return to citation in text: [1] -
Gholampour, N.; Yusubov, M.; Verpoort, F. Catal. Rev.: Sci. Eng. 2016, 58, 113–156. doi:10.1080/01614940.2015.1100871
Return to citation in text: [1] -
Lwin, S.; Wachs, I. E. ACS Catal. 2014, 4, 2505–2520. doi:10.1021/cs500528h
Return to citation in text: [1] [2] [3] [4] -
Amakawa, K.; Kröhnert, J.; Wrabetz, S.; Frank, B.; Hemmann, F.; Jäger, C.; Schlögl, R.; Trunschke, A. ChemCatChem 2015, 7, 4059–4065. doi:10.1002/cctc.201500725
Return to citation in text: [1] [2] -
Balcar, H.; Čejka, J. Coord. Chem. Rev. 2013, 257, 3107–3124. doi:10.1016/j.ccr.2013.07.026
Return to citation in text: [1] -
Balcar, H.; Mishra, D.; Marceau, E.; Carrier, X.; Žilková, N.; Bastl, Z. Appl. Catal., A 2009, 359, 129–135. doi:10.1016/j.apcata.2009.02.037
Return to citation in text: [1] [2] [3] [4] [5] -
Topka, P.; Balcar, H.; Rathouský, J.; Žilková, N.; Verpoort, F.; Čejka, J. Microporous Mesoporous Mater. 2006, 96, 44–54. doi:10.1016/j.micromeso.2006.06.016
Return to citation in text: [1] [2] [3] [4] -
Lin, B.; Zhang, Q.; Wang, Y. Ind. Eng. Chem. Res. 2009, 48, 10788–10795. doi:10.1021/ie901227p
Return to citation in text: [1] -
Handzlik, J. J. Mol. Catal. A: Chem. 2010, 316, 106–111. doi:10.1016/j.molcata.2009.10.007
Return to citation in text: [1] [2] -
Li, X.; Zhang, W.; Liu, S.; Han, X.; Xu, L.; Bao, X. J. Mol. Catal. A: Chem. 2006, 250, 94–99. doi:10.1016/j.molcata.2006.01.046
Return to citation in text: [1] -
Díaz, U.; Corma, A. Dalton Trans. 2014, 43, 10292–10316. doi:10.1039/c3dt53181c
Return to citation in text: [1] -
Roth, W. J.; Nachtigall, P.; Morris, R. E.; Čejka, J. Chem. Rev. 2014, 114, 4807–4837. doi:10.1021/cr400600f
Return to citation in text: [1] -
Wei, R.; Yang, H.; Scott, J. A.; Aguey-Zinsou, K.-F.; Zhang, D. Mater. Today Chem. 2018, 8, 1–12. doi:10.1016/j.mtchem.2018.01.002
Return to citation in text: [1] -
Opanasenko, M. V.; Roth, W. J.; Čejka, J. Catal. Sci. Technol. 2016, 6, 2467–2484. doi:10.1039/c5cy02079d
Return to citation in text: [1] -
Juttu, G. G.; Lobo, R. F. Microporous Mesoporous Mater. 2000, 40, 9–23. doi:10.1016/s1387-1811(00)00233-x
Return to citation in text: [1] [2] [3] -
Roth, W. J.; Čejka, J.; Millini, R.; Montanari, E.; Gil, B.; Kubu, M. Chem. Mater. 2015, 27, 4620–4629. doi:10.1021/acs.chemmater.5b01030
Return to citation in text: [1] -
Leonowicz, M. E.; Lawton, J. A.; Lawton, S. L.; Rubin, M. K. Science 1994, 264, 1910–1913. doi:10.1126/science.264.5167.1910
Return to citation in text: [1] -
Choi, M.; Na, K.; Kim, J.; Sakamoto, Y.; Terasaki, O.; Ryoo, R. Nature 2009, 461, 246–249. doi:10.1038/nature08288
Return to citation in text: [1] [2] -
Přech, J.; Pizarro, P.; Serrano, D. P.; Čejka, J. Chem. Soc. Rev. 2018, 47, 8263–8306. doi:10.1039/c8cs00370j
Return to citation in text: [1] -
Liu, S.; Li, X.; Xin, W.; Xie, S.; Zeng, P.; Zhang, L.; Xu, L. J. Nat. Gas Chem. 2010, 19, 482–486. doi:10.1016/s1003-9953(09)60095-5
Return to citation in text: [1] -
Balcar, H.; Žilková, N.; Kubů, M.; Mazur, M.; Bastl, Z.; Čejka, J. Beilstein J. Org. Chem. 2015, 11, 2087–2096. doi:10.3762/bjoc.11.225
Return to citation in text: [1] [2] [3] -
Bordiga, S.; Lamberti, C.; Bonino, F.; Travert, A.; Thibault-Starzyk, F. Chem. Soc. Rev. 2015, 44, 7262–7341. doi:10.1039/c5cs00396b
Return to citation in text: [1] -
Topka, P. Molybdenum oxide supported on mesoporous molecular sieves – new catalysts for alkene metathesis and alkyne polymerization. Ph.D. Thesis, Charles University, Prague, 2008.
Return to citation in text: [1] -
Handzlik, J.; Ogonowski, J.; Stoch, J.; Mikołajczyk, M.; Michorczyk, P. Appl. Catal., A 2006, 312, 213–219. doi:10.1016/j.apcata.2006.07.002
Return to citation in text: [1] -
Lim, T. H.; Nam, K.; Song, I. K.; Lee, K.-Y.; Kim, D. H. Appl. Catal., A 2018, 552, 11–20. doi:10.1016/j.apcata.2017.12.021
Return to citation in text: [1] -
Zhao, P.; Ye, L.; Sun, Z.; Lo, B. T. W.; Woodcock, H.; Huang, C.; Tang, C.; Kirkland, A. I.; Mei, D.; Edman Tsang, S. C. J. Am. Chem. Soc. 2018, 140, 6661–6667. doi:10.1021/jacs.8b03012
Return to citation in text: [1] -
Knifton, J. F.; Sanderson, J. R.; Dai, P. E. Catal. Lett. 1994, 28, 223–230. doi:10.1007/bf00806051
Return to citation in text: [1] -
O'Connor, C. T.; Kojima, M. Catal. Today 1990, 6, 329–349. doi:10.1016/0920-5861(90)85008-c
Return to citation in text: [1] -
Kresge, C. T.; Roth, W. J.; Simmons, K. G.; Vartuli, J. C. Crystalline oxide material. U.S. Patent 5,229,341, June 20, 1993.
Return to citation in text: [1] -
Fung, A. S.; Lawton, S. L.; Roth, W. J. Synthetic layered MCM-56, its synthesis and use. U. S. Patent 5,362,697, Nov 8, 1994.
Return to citation in text: [1] -
Emeis, C. A. J. Catal. 1993, 141, 347–354. doi:10.1006/jcat.1993.1145
Return to citation in text: [1]
1. | Ivin, K. J.; Mol, J. C. Applications of the Olefin Metathesis Reaction. Olefin Metathesis and Metathesis Polymerization, 2nd ed.; Academic Press: London, 1997; pp 397–410. doi:10.1016/b978-012377045-5/50018-5 |
8. | Balcar, H.; Čejka, J. Coord. Chem. Rev. 2013, 257, 3107–3124. doi:10.1016/j.ccr.2013.07.026 |
9. | Balcar, H.; Mishra, D.; Marceau, E.; Carrier, X.; Žilková, N.; Bastl, Z. Appl. Catal., A 2009, 359, 129–135. doi:10.1016/j.apcata.2009.02.037 |
10. | Topka, P.; Balcar, H.; Rathouský, J.; Žilková, N.; Verpoort, F.; Čejka, J. Microporous Mesoporous Mater. 2006, 96, 44–54. doi:10.1016/j.micromeso.2006.06.016 |
9. | Balcar, H.; Mishra, D.; Marceau, E.; Carrier, X.; Žilková, N.; Bastl, Z. Appl. Catal., A 2009, 359, 129–135. doi:10.1016/j.apcata.2009.02.037 |
26. | Topka, P. Molybdenum oxide supported on mesoporous molecular sieves – new catalysts for alkene metathesis and alkyne polymerization. Ph.D. Thesis, Charles University, Prague, 2008. |
6. | Lwin, S.; Wachs, I. E. ACS Catal. 2014, 4, 2505–2520. doi:10.1021/cs500528h |
7. | Amakawa, K.; Kröhnert, J.; Wrabetz, S.; Frank, B.; Hemmann, F.; Jäger, C.; Schlögl, R.; Trunschke, A. ChemCatChem 2015, 7, 4059–4065. doi:10.1002/cctc.201500725 |
9. | Balcar, H.; Mishra, D.; Marceau, E.; Carrier, X.; Žilková, N.; Bastl, Z. Appl. Catal., A 2009, 359, 129–135. doi:10.1016/j.apcata.2009.02.037 |
10. | Topka, P.; Balcar, H.; Rathouský, J.; Žilková, N.; Verpoort, F.; Čejka, J. Microporous Mesoporous Mater. 2006, 96, 44–54. doi:10.1016/j.micromeso.2006.06.016 |
6. | Lwin, S.; Wachs, I. E. ACS Catal. 2014, 4, 2505–2520. doi:10.1021/cs500528h |
7. | Amakawa, K.; Kröhnert, J.; Wrabetz, S.; Frank, B.; Hemmann, F.; Jäger, C.; Schlögl, R.; Trunschke, A. ChemCatChem 2015, 7, 4059–4065. doi:10.1002/cctc.201500725 |
9. | Balcar, H.; Mishra, D.; Marceau, E.; Carrier, X.; Žilková, N.; Bastl, Z. Appl. Catal., A 2009, 359, 129–135. doi:10.1016/j.apcata.2009.02.037 |
2. | Hahn, T.; Bentrup, U.; Armbrüster, M.; Kondratenko, E. V.; Linke, D. ChemCatChem 2014, 6, 1664–1672. doi:10.1002/cctc.201400040 |
3. | Zhang, D.; Li, X.; Liu, S.; Huang, S.; Zhu, X.; Chen, F.; Xie, S.; Xu, L. Appl. Catal., A 2012, 439-440, 171–178. doi:10.1016/j.apcata.2012.07.002 |
4. | Hahn, T.; Kondratenko, E. V.; Linke, D. Chem. Commun. 2014, 50, 9060–9063. doi:10.1039/c4cc01827c |
5. | Gholampour, N.; Yusubov, M.; Verpoort, F. Catal. Rev.: Sci. Eng. 2016, 58, 113–156. doi:10.1080/01614940.2015.1100871 |
25. | Bordiga, S.; Lamberti, C.; Bonino, F.; Travert, A.; Thibault-Starzyk, F. Chem. Soc. Rev. 2015, 44, 7262–7341. doi:10.1039/c5cs00396b |
22. | Přech, J.; Pizarro, P.; Serrano, D. P.; Čejka, J. Chem. Soc. Rev. 2018, 47, 8263–8306. doi:10.1039/c8cs00370j |
24. | Balcar, H.; Žilková, N.; Kubů, M.; Mazur, M.; Bastl, Z.; Čejka, J. Beilstein J. Org. Chem. 2015, 11, 2087–2096. doi:10.3762/bjoc.11.225 |
18. | Juttu, G. G.; Lobo, R. F. Microporous Mesoporous Mater. 2000, 40, 9–23. doi:10.1016/s1387-1811(00)00233-x |
19. | Roth, W. J.; Čejka, J.; Millini, R.; Montanari, E.; Gil, B.; Kubu, M. Chem. Mater. 2015, 27, 4620–4629. doi:10.1021/acs.chemmater.5b01030 |
20. | Leonowicz, M. E.; Lawton, J. A.; Lawton, S. L.; Rubin, M. K. Science 1994, 264, 1910–1913. doi:10.1126/science.264.5167.1910 |
21. | Choi, M.; Na, K.; Kim, J.; Sakamoto, Y.; Terasaki, O.; Ryoo, R. Nature 2009, 461, 246–249. doi:10.1038/nature08288 |
9. | Balcar, H.; Mishra, D.; Marceau, E.; Carrier, X.; Žilková, N.; Bastl, Z. Appl. Catal., A 2009, 359, 129–135. doi:10.1016/j.apcata.2009.02.037 |
10. | Topka, P.; Balcar, H.; Rathouský, J.; Žilková, N.; Verpoort, F.; Čejka, J. Microporous Mesoporous Mater. 2006, 96, 44–54. doi:10.1016/j.micromeso.2006.06.016 |
24. | Balcar, H.; Žilková, N.; Kubů, M.; Mazur, M.; Bastl, Z.; Čejka, J. Beilstein J. Org. Chem. 2015, 11, 2087–2096. doi:10.3762/bjoc.11.225 |
14. | Díaz, U.; Corma, A. Dalton Trans. 2014, 43, 10292–10316. doi:10.1039/c3dt53181c |
15. | Roth, W. J.; Nachtigall, P.; Morris, R. E.; Čejka, J. Chem. Rev. 2014, 114, 4807–4837. doi:10.1021/cr400600f |
16. | Wei, R.; Yang, H.; Scott, J. A.; Aguey-Zinsou, K.-F.; Zhang, D. Mater. Today Chem. 2018, 8, 1–12. doi:10.1016/j.mtchem.2018.01.002 |
17. | Opanasenko, M. V.; Roth, W. J.; Čejka, J. Catal. Sci. Technol. 2016, 6, 2467–2484. doi:10.1039/c5cy02079d |
11. | Lin, B.; Zhang, Q.; Wang, Y. Ind. Eng. Chem. Res. 2009, 48, 10788–10795. doi:10.1021/ie901227p |
12. | Handzlik, J. J. Mol. Catal. A: Chem. 2010, 316, 106–111. doi:10.1016/j.molcata.2009.10.007 |
13. | Li, X.; Zhang, W.; Liu, S.; Han, X.; Xu, L.; Bao, X. J. Mol. Catal. A: Chem. 2006, 250, 94–99. doi:10.1016/j.molcata.2006.01.046 |
23. | Liu, S.; Li, X.; Xin, W.; Xie, S.; Zeng, P.; Zhang, L.; Xu, L. J. Nat. Gas Chem. 2010, 19, 482–486. doi:10.1016/s1003-9953(09)60095-5 |
28. | Lim, T. H.; Nam, K.; Song, I. K.; Lee, K.-Y.; Kim, D. H. Appl. Catal., A 2018, 552, 11–20. doi:10.1016/j.apcata.2017.12.021 |
12. | Handzlik, J. J. Mol. Catal. A: Chem. 2010, 316, 106–111. doi:10.1016/j.molcata.2009.10.007 |
27. | Handzlik, J.; Ogonowski, J.; Stoch, J.; Mikołajczyk, M.; Michorczyk, P. Appl. Catal., A 2006, 312, 213–219. doi:10.1016/j.apcata.2006.07.002 |
32. | Kresge, C. T.; Roth, W. J.; Simmons, K. G.; Vartuli, J. C. Crystalline oxide material. U.S. Patent 5,229,341, June 20, 1993. |
33. | Fung, A. S.; Lawton, S. L.; Roth, W. J. Synthetic layered MCM-56, its synthesis and use. U. S. Patent 5,362,697, Nov 8, 1994. |
21. | Choi, M.; Na, K.; Kim, J.; Sakamoto, Y.; Terasaki, O.; Ryoo, R. Nature 2009, 461, 246–249. doi:10.1038/nature08288 |
24. | Balcar, H.; Žilková, N.; Kubů, M.; Mazur, M.; Bastl, Z.; Čejka, J. Beilstein J. Org. Chem. 2015, 11, 2087–2096. doi:10.3762/bjoc.11.225 |
30. | Knifton, J. F.; Sanderson, J. R.; Dai, P. E. Catal. Lett. 1994, 28, 223–230. doi:10.1007/bf00806051 |
31. | O'Connor, C. T.; Kojima, M. Catal. Today 1990, 6, 329–349. doi:10.1016/0920-5861(90)85008-c |
18. | Juttu, G. G.; Lobo, R. F. Microporous Mesoporous Mater. 2000, 40, 9–23. doi:10.1016/s1387-1811(00)00233-x |
18. | Juttu, G. G.; Lobo, R. F. Microporous Mesoporous Mater. 2000, 40, 9–23. doi:10.1016/s1387-1811(00)00233-x |
29. | Zhao, P.; Ye, L.; Sun, Z.; Lo, B. T. W.; Woodcock, H.; Huang, C.; Tang, C.; Kirkland, A. I.; Mei, D.; Edman Tsang, S. C. J. Am. Chem. Soc. 2018, 140, 6661–6667. doi:10.1021/jacs.8b03012 |
6. | Lwin, S.; Wachs, I. E. ACS Catal. 2014, 4, 2505–2520. doi:10.1021/cs500528h |
10. | Topka, P.; Balcar, H.; Rathouský, J.; Žilková, N.; Verpoort, F.; Čejka, J. Microporous Mesoporous Mater. 2006, 96, 44–54. doi:10.1016/j.micromeso.2006.06.016 |
© 2018 Balcar et al.; licensee Beilstein-Institut.
This is an Open Access article under the terms of the Creative Commons Attribution License (http://creativecommons.org/licenses/by/4.0). Please note that the reuse, redistribution and reproduction in particular requires that the authors and source are credited.
The license is subject to the Beilstein Journal of Organic Chemistry terms and conditions: (https://www.beilstein-journals.org/bjoc)