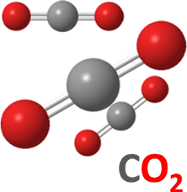
Graphical Abstract
It is our pleasure to introduce this Thematic Series on CO2 chemistry for the Beilstein Journal of Organic Chemistry (BJOC). Today’s growing demand for energy, materials and chemicals has prompted renewed interest in CO2 chemistry. More resource-efficient chemical processes are being implemented, while we are facing the change from a fossil fuel-based society to one that must rely on the sustainable use of renewable resources. Although there are many ways to harness renewable energy resources, much of the needed materials and chemicals will continue to be carbon-based.
One of the most abundant renewable resources of carbon is carbon dioxide (Figure 1). Carbon capture technologies are being implemented [1] to capture a part of the yearly anthropogenic CO2 emission of 36,600 million metric tons of CO2 [2]. If only a fraction of the captured CO2 stream could be made available for chemical production, a significant contribution to the annual production of carbon-based materials and chemicals could be supplied. Here, we offer the reader to relate these figures with the annual production of polymeric materials of 280 million metric tons [3]. Remarkably, 110 million metric tons of CO2 per year for producing urea, methanol and salicylic acid are industrial reality today. These applications clearly illustrate the path forward. Due to the abundant availability of pure CO2 gas streams [1], it is only logical to promote a more widespread use of carbon dioxide as chemical feedstock. Notably, the use of CO2 for manufacturing materials and chemicals is still in its infancy.
Carbon dioxide (CO2) has long stirred the fascination of chemists. A rich chemistry has evolved utilizing this molecule in chemical synthesis [4]. Hitherto the low reactivity of the CO2 molecule poses significant challenges to the utilization of carbon dioxide in industrial applications. Thus, the CO2 molecule is commonly perceived to be highly inert. This perception clearly stems from the high chemical stability of carbon dioxide. However, the reactivity of the CO2 molecule may be underestimated. Carbon dioxide is isoelectronic to highly reactive molecules such as isocyanates and ketenes (Figure 2). This implies that reactivity and kinetic limitations may be encountered much less frequently in the chemical conversion of carbon dioxide than generally assumed.
Figure 2: Examples of highly reactive molecules that are isoelectronic to carbon dioxide.
Figure 2: Examples of highly reactive molecules that are isoelectronic to carbon dioxide.
To overcome its thermodynamically low level, additional energy is required to activate the CO2 molecule. The threefold reactivity (Figure 3) of CO2 with a nucleophilic oxygen atom, an electrophilic carbon atom and a π system provides the chemist with many options. Likewise, a rich coordination chemistry to metal centres has been reported for CO2 [5,6]. A forthcoming path is the reaction of CO2 to form energy-rich intermediates that can subsequently transfer the CO2 molecule to target substrates [7]. The use of efficient catalysts is often another requisite to direct the reaction pathways with high selectivity to yield the desired target products and to overcome kinetic limitations associated with certain slow elementary steps.
![[1860-5397-11-76-3]](/bjoc/content/figures/1860-5397-11-76-3.png?scale=2.0&max-width=1024&background=FFFFFF)
Figure 3: Threefold reactivity of carbon dioxide and examples for different activation modes for CO2 involving metal centres in homogeneous and heterogeneous catalysts [5,6].
Figure 3: Threefold reactivity of carbon dioxide and examples for different activation modes for CO2 involvin...
This Thematic Series on CO2 chemistry presents intriguing approaches regarding different methodologies to activate carbon dioxide. One emerging field is the electrochemical fixation of CO2, which can be applied in the synthesis of carboxylic acids [8]. Also highly interesting is the combination of enzymatic and photocatalytic approaches for activating CO2 [9]. Bifunctional catalyst systems are frequently needed and well-understood in the synthesis of cyclic carbonates [10]. Activation of carbon dioxide by inserting it into metal-alkoxide bonds allows for subsequent applications in polymer synthesis such as the copolymerisation of carbon dioxide with epoxides and other co-monomers [11]. Here, the catalysis with cobalt complexes still presents surprising effects [12]. More efficient systems for CO2 capture are being developed on the basis of amine-functionalised ionic liquids where zwitterionic adduct formation is the key to higher efficiency [13]. Furthermore, many physical properties of carbon dioxide are outstanding, making supercritical carbon dioxide a solvent like no other [14].
Altogether, the articles in this Thematic Series present a remarkable overview of opportunities in the field of CO2 chemistry from many of its top practitioners. These opportunities are harbingers of the many additional reactions, reactivity modes and catalysts that remain to be discovered. Exploiting carbon dioxide to create economic value will be the driving force for the more widespread use of this fascinating molecule. In the long term, we envision mankind creating an anthropogenic carbon loop where CO2 released at the end of the life span of carbon-based goods of everyday life is again employed in the production of new materials and chemicals.
We are highly grateful to the authors for their excellent contributions towards making this Thematic Series as successful as the previous editions.
Thomas E. Müller and Walter Leitner
Aachen, April 2015
References
-
Markewitz, P.; Kuckshinrichs, W.; Leitner, W.; Linssen, J.; Zapp, P.; Bongartz, R.; Schreiber, A.; Müller, T. E. Energy Environ. Sci. 2012, 5, 7281. doi:10.1039/c2ee03403d
Return to citation in text: [1] [2] -
Canadell, J. G.; Le Quére, C.; Raupach, M. R.; Field, C. B.; Buitenhuis, E. T.; Ciais, P.; Conway, T. J.; Gillett, N. P.; Houghton, R. A.; Marland, G. Proc. Natl. Acad. Sci. U. S. A. 2007, 104, 18866–18870. doi:10.1073/pnas.0702737104
Return to citation in text: [1] -
Plastics - The Facts; PlasticsEurope Association of Plastics Manufacturers: Brussels, Belgium, 2012.
Return to citation in text: [1] -
Peters, M.; Köhler, B.; Kuckshinrichs, W.; Leitner, W.; Markewitz, P.; Müller, T. E. ChemSusChem 2011, 4, 1216. doi:10.1002/cssc.201000447
Return to citation in text: [1] -
Chiesa, M.; Giamello, E. Chem. – Eur. J. 2007, 13, 1261. doi:10.1002/chem.200600792
Return to citation in text: [1] [2] -
Gibson, D. H. Chem. Rev. 1996, 96, 2063. doi:10.1021/cr940212c
Return to citation in text: [1] [2] -
Elmas, S.; Subhani, M. A.; Vogt, H.; Leitner, W.; Müller, T. E. Green Chem. 2013, 15, 1356. doi:10.1039/c3gc40147b
Return to citation in text: [1] -
Matthessen, R.; Fransaer, J.; Binnemans, K.; De Vos, D. E. Beilstein J. Org. Chem. 2014, 10, 2484. doi:10.3762/bjoc.10.260
Return to citation in text: [1] -
Aresta, M.; Dibenedetto, A.; Baran, T.; Angelini, A.; Łabuz, P.; Macyk, W. Beilstein J. Org. Chem. 2014, 10, 2556. doi:10.3762/bjoc.10.267
Return to citation in text: [1] -
Martín, C.; Kleij, A. W. Beilstein J. Org. Chem. 2014, 10, 1817. doi:10.3762/bjoc.10.191
Return to citation in text: [1] -
Jeon, J. Y.; Eo, S. C.; Varghese, J. K.; Lee, B. Y. Beilstein J. Org. Chem. 2014, 10, 1787. doi:10.3762/bjoc.10.187
Return to citation in text: [1] -
Elmas, S.; Subhani, M. A.; Leitner, W.; Müller, T. E. Beilstein J. Org. Chem. 2015, 11, 42. doi:10.3762/bjoc.11.7
Return to citation in text: [1] -
Yang, Z.-Z.; He, L.-N. Beilstein J. Org. Chem. 2014, 10, 1959. doi:10.3762/bjoc.10.204
Return to citation in text: [1] -
Peach, J.; Eastoe, J. Beilstein J. Org. Chem. 2014, 10, 1878. doi:10.3762/bjoc.10.196
Return to citation in text: [1]
1. | Markewitz, P.; Kuckshinrichs, W.; Leitner, W.; Linssen, J.; Zapp, P.; Bongartz, R.; Schreiber, A.; Müller, T. E. Energy Environ. Sci. 2012, 5, 7281. doi:10.1039/c2ee03403d |
4. | Peters, M.; Köhler, B.; Kuckshinrichs, W.; Leitner, W.; Markewitz, P.; Müller, T. E. ChemSusChem 2011, 4, 1216. doi:10.1002/cssc.201000447 |
14. | Peach, J.; Eastoe, J. Beilstein J. Org. Chem. 2014, 10, 1878. doi:10.3762/bjoc.10.196 |
1. | Markewitz, P.; Kuckshinrichs, W.; Leitner, W.; Linssen, J.; Zapp, P.; Bongartz, R.; Schreiber, A.; Müller, T. E. Energy Environ. Sci. 2012, 5, 7281. doi:10.1039/c2ee03403d |
3. | Plastics - The Facts; PlasticsEurope Association of Plastics Manufacturers: Brussels, Belgium, 2012. |
12. | Elmas, S.; Subhani, M. A.; Leitner, W.; Müller, T. E. Beilstein J. Org. Chem. 2015, 11, 42. doi:10.3762/bjoc.11.7 |
2. | Canadell, J. G.; Le Quére, C.; Raupach, M. R.; Field, C. B.; Buitenhuis, E. T.; Ciais, P.; Conway, T. J.; Gillett, N. P.; Houghton, R. A.; Marland, G. Proc. Natl. Acad. Sci. U. S. A. 2007, 104, 18866–18870. doi:10.1073/pnas.0702737104 |
13. | Yang, Z.-Z.; He, L.-N. Beilstein J. Org. Chem. 2014, 10, 1959. doi:10.3762/bjoc.10.204 |
8. | Matthessen, R.; Fransaer, J.; Binnemans, K.; De Vos, D. E. Beilstein J. Org. Chem. 2014, 10, 2484. doi:10.3762/bjoc.10.260 |
10. | Martín, C.; Kleij, A. W. Beilstein J. Org. Chem. 2014, 10, 1817. doi:10.3762/bjoc.10.191 |
5. | Chiesa, M.; Giamello, E. Chem. – Eur. J. 2007, 13, 1261. doi:10.1002/chem.200600792 |
6. | Gibson, D. H. Chem. Rev. 1996, 96, 2063. doi:10.1021/cr940212c |
11. | Jeon, J. Y.; Eo, S. C.; Varghese, J. K.; Lee, B. Y. Beilstein J. Org. Chem. 2014, 10, 1787. doi:10.3762/bjoc.10.187 |
7. | Elmas, S.; Subhani, M. A.; Vogt, H.; Leitner, W.; Müller, T. E. Green Chem. 2013, 15, 1356. doi:10.1039/c3gc40147b |
5. | Chiesa, M.; Giamello, E. Chem. – Eur. J. 2007, 13, 1261. doi:10.1002/chem.200600792 |
6. | Gibson, D. H. Chem. Rev. 1996, 96, 2063. doi:10.1021/cr940212c |
9. | Aresta, M.; Dibenedetto, A.; Baran, T.; Angelini, A.; Łabuz, P.; Macyk, W. Beilstein J. Org. Chem. 2014, 10, 2556. doi:10.3762/bjoc.10.267 |
© 2015 Müller and Leitner; licensee Beilstein-Institut.
This is an Open Access article under the terms of the Creative Commons Attribution License (http://creativecommons.org/licenses/by/2.0), which permits unrestricted use, distribution, and reproduction in any medium, provided the original work is properly cited.
The license is subject to the Beilstein Journal of Organic Chemistry terms and conditions: (http://www.beilstein-journals.org/bjoc)