Abstract
Novel pyridine-based fluorescing compounds, viz. pyrido[1,2-a]pyrrolo[3,4-d]pyrimidines 3a,b and N-methyl-4-((pyridin-2-yl)amino)maleimides 4a–e, were selectively prepared by a one-pot reaction between a functionalized maleimide and 2-aminopyridines with electron-donating or electron-withdrawing groups at position 5 and were investigated photophysically and computationally. The photophysical studies revealed that all the synthesized compounds exhibited fluorescence in organic solvents, while N-methyl-4-((pyridin-2-yl)amino)-substituted maleimide derivatives 4a–e, which are based on an acceptor–donor–acceptor (A–D–A) system, exhibited aggregation-induced emission enhancement (AIEE) properties in aqueous media. Compounds 4a and 4e, bearing electron-withdrawing groups (Br and CF3, respectively) showed 7.0 and 15 times fluorescence enhancement. Time-dependent density functional theory (TD-DFT) calculations were performed to gain better insight into the electronic nature of the compounds with and without AIEE properties.
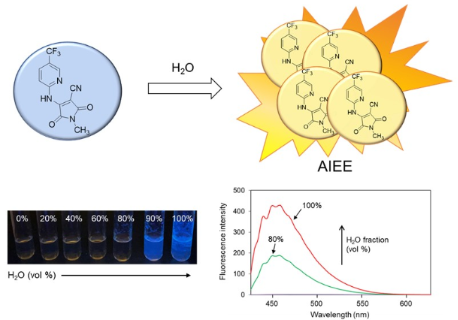
Graphical Abstract
Introduction
Fluorescent compounds have attracted considerable attention as functional materials because of their applications in areas such as information devices, displays, and clinical diagnosis [1-3]. Organic compounds with planar structures and large π systems exhibit strong fluorescence in dilute solutions [4,5]. However, these compounds usually form aggregate structures in high-concentration solutions, and their emission efficiency, chromogenic properties, and light sensitivity decrease rapidly [5,6]. In recent years, contrary to conventional fluorescent compounds, aggregation-induced emission enhancement (AIEE)-based compounds that exhibit strong fluorescence in aggregate structures have been reported [7-10]. Because the aggregated state of AIEE-based compounds is affected by the external environment, these compounds have found use in clinical applications as chemical sensors or fluorescent probes [7-10].
Pyridine is a nitrogen-containing heterocyclic compound found in many bioactive substances and medicines as one of the basic core skeletons [11,12]. In addition, pyridine is an essential skeleton for fluorescent compounds, and fluorescence can be enhanced by optimizing the internal charge transfer (ICT) state of pyridine by introducing electron-donating or electron-withdrawing groups [13-15]. Previously, we have reported various pyridine derivatives, including polysubstituted pyridines and fused pyridines, which exhibited strong fluorescence in organic solvents (ethanol and dichloromethane) [16-19], while their fluorescence in aqueous media was quenched due to aggregation-caused quenching (ACQ) [10]. Generally, the restriction of intermolecular π–π interactions in highly planar compounds plays a key role in aggregate structures exhibiting fluorescence [5,6,10]. In addition, intramolecular mechanisms, such as intramolecular rotation (RIR), intramolecular charge transfer, and twisted intramolecular charge transfer (TICT) are involved in AIEE [20-22]. Various AIEE-based luminogens have been developed based on these mechanisms; however, many of them are high-molecular-weight compounds (MW > 500) with bulky substituents, which limits their clinical applications such as cell imaging and cell sorting.
To develop low-molecular-weight AIEE-based luminogens, we have synthesized a series of fluorescent compounds by the reaction of nucleophilic maleimides with 2-aminopyridines. This resulted in the development of a novel method to obtain heterocyclic compounds, such as ring-fused pyridines (pyrido[1,2-a]pyrrolo[3,4-d]pyrimidines) and secondary aminopyridines (N-methyl-4-((pyridin-2-yl)amino)-substituted maleimides), by changing the substituents at position 5 of the 2-aminopyridine. Interestingly, among these pyridine derivatives, secondary aminopyridines based on the acceptor–donor–acceptor (A–D–A) system exhibit AIEE properties in aqueous media, which may be novel candidate molecules for AIEE. Herein, we report the synthesis, photophysical properties, and computational studies of pyrido[1,2-a]pyrrolo[3,4-d]pyrimidines and N-methyl-4-((pyridin-2-yl)amino)-substituted maleimides.
Results and Discussion
Maleimides are versatile reagents for the synthesis of heterocyclic compounds. Previously, we have reported functional maleimides derived from ketene dithioacetals and have prepared fluorescent compounds using this reagent [23,24]. In this study, we used 1-methyl-4-(methylsulfanyl)-2,5-dioxo-2,5-dihydro-1H-pyrrole-3-carbonitrile (1) with a methylsulfanyl group as a good leaving group. As shown in Scheme 1, the one-pot reaction of 1 with 2-aminopyridine (2a) proceeded by refluxing in ethanol for 2 h to produce the ring-fused pyridine compound, 10-imino-2-methylpyrido[1,2-a]pyrrolo[3,4-d]pyrimidine-1,3(2H,10H)-dione (3a), in 97% yield. The chemical structure of product 3a was confirmed by 1H and 13C NMR spectroscopy (Figures S1 and S2 in Supporting Information File 1) and was obtained via the following reaction mechanism: nucleophilic attack of the amino group of 2-aminopyridine to maleimide 1, followed by elimination of the methylsulfanyl group, and subsequent cyclization (Figure 1). The ring-fused pyridine compound 3b was obtained from the reaction of 1 with 2-aminopyridine 2b, which has an electron-donating methyl group at position 5 of the pyridine ring (Table 1). On the other hand, the reaction of 1 with 5-bromo-2-aminopyridine (2c) afforded an N-methyl-4-((pyridin-2-yl)amino)-substitued maleimide, 4-((5-bromopyridin-2-yl)amino)-1-methyl-2,5-dioxo-2,5-dihydro-1H-pyrrole-3-carbonitrile (4a), based on an A–D–A system containing two acceptor (maleimide and pyridine) and one donor (secondary amine) moieties in 72% yield (Scheme 1). Due to the electron-withdrawing effect of the bromo substituent at position 5 of the pyridine ring, the cyclization reaction did not occur. The structure of product 4a was confirmed by 1H and 13C NMR spectroscopy (Figures S5 and S6 in Supporting Information File 1). Similarly, the reaction of 1 with 2-aminopyridine derivatives 2d–g bearing electron-withdrawing groups, except for the nitro-group containing substrate 2h, at position 5 of the pyridine ring, afforded N-methyl-4-((pyridin-2-yl)amino)-substituted maleimide derivatives 4b–e (Table 1).
Scheme 1: Syntheses of pyrido[1,2-a]pyrrolo[3,4-d]pyrimidine 3a and N-methyl-4-((5-bromopyridin-2-yl)amino)-substituted maleimide 4a.
Scheme 1: Syntheses of pyrido[1,2-a]pyrrolo[3,4-d]pyrimidine 3a and N-methyl-4-((5-bromopyridin-2-yl)amino)-s...
Figure 1: Presumed reaction mechanism to produce 3a.
Figure 1: Presumed reaction mechanism to produce 3a.
Table 1: Syntheses of pyrido[1,2-a]pyrrolo[3,4-d]pyrimidines 3a,b and N-methyl-4-((pyridin-2-yl)amino)-substituted maleimide derivatives 4a–e.
|
||||
entry | 2-aminopyridine | R | yield (%) | product |
1 | 2a | 5-H | 97 | 3a |
2 | 2b | 5-CH3 | 86 | 3b |
3 | 2c | 5-Br | 72 | 4a |
4 | 2d | 5-F | 68 | 4b |
5 | 2e | 5-CN | 32 | 4c |
6 | 2f | 5-COOCH3 | 43 | 4d |
7 | 2g | 5-CF3 | 46 | 4e |
8 | 2h | 5-NO2 | – | no reaction |
Naka et al. previously reported the facile syntheses of N-alkyl-arylaminomaleimide derivatives as D–A system molecules by the reaction of dimethyl acetylenedicarboxylate with arylamines, which showed AIEE properties in 10% or 20% (v/v) THF aqueous solution [25]. In this reaction, the same aryl groups were easily introduced to the 4 and N-positions of maleimide. However, an additional step was required to synthesize N-alkyl-arylaminomaleimides bearing different aryl and alkyl groups. In addition, the synthesis of A–D–A-type molecules such as the introduction of pyridine rings has not been examined. Our simple one-pot method easily enables the introduction of a pyridine group to maleimide under mild conditions, affording the A–D–A-type molecules that are expected to be novel low-molecular-weight fluorescent materials in moderately good yields.
The UV–vis spectra of all compounds were recorded in ethanol (EtOH), a polar solvent, and in dichloromethane (DCM), a non-polar solvent (see Figures S15–S17 in Supporting Information File 1). The maximum absorption peaks (λmax) shifted slightly to longer wavelengths in dichloromethane. Table 2 summarizes the excitation maxima (Exmax), emission maxima (Emmax), and fluorescence quantum yields (Φ) of the molecules from fluorescence spectroscopic studies. The pyrido[1,2-a]pyrrolo[3,4-d]pyrimidine derivatives 3a,b possessing a highly complex ring-fused system emitted fluorescence at 545–546 nm and 537–538 nm in ethanol and dichloromethane (Figures S18 and S19 in Supporting Information File 1). The Φ value of 3b increased with the introduction of a methyl group at position 5 of the pyridine ring of 3a in both solvents, suggesting that the electron-donating effect of the methyl group stabilized the ring system and thus induced increased fluorescence. The N-methyl-4-((pyridin-2-yl)amino)-substituted maleimides 4a–e comprised an A–D–A system, which exhibited an obvious substitution effect on the fluorescence properties (Figures S18 and S19 in Supporting Information File 1). Although the unsubstituted compound 4a emitted weak fluorescence at 553 nm and 539 nm in ethanol and dichloromethane, respectively, the introduction of electron-withdrawing groups at position 5 of the pyridine ring of 4a greatly affected the Exmax value of products 4b–e and induced hypsochromic shifts of about 72–82 nm in ethanol. In contrast, similar hypsochromic shifts were observed in dichloromethane only in case of compounds 4c and 4e bearing strong electron-withdrawing substituents (CN and CF3, respectively), and the Φ value of these compounds increased. These solvatochromic effects could be attributed to changes in the ICT state of the molecules, indicating that fluorescence properties of N-methyl-4-((pyridin-2-yl)amino)-substituted maleimides could be modulated by the electron push–pull effect of substituents.
Table 2: Fluorescence data for products 3a,b, and 4a–e in EtOH and DCM.
dissolved in EtOH | dissolved in DCM | ||||||
compound | EXmax (nm)a | EMmax (nm)b | Φc | EXmax (nm)a | EMmax (nm)b | Φc | |
3a | 468 | 545 | 0.01 | 471 | 537 | 0.03 | |
3b | 469 | 546 | 0.02 | 481 | 538 | 0.06 | |
4a | 476 | 553 | 0.01 | 477 | 539 | 0.01 | |
4b | 415 | 471 | 0.01 | 474 | 538 | 0.01 | |
4c | 377 | 481 | 0.01< | 373 | 469 | 0.03 | |
4d | 378 | 481 | 0.01< | 475 | 539 | 0.01< | |
4e | 378 | 477 | 0.01 | 373 | 470 | 0.02 |
aEach excitation wavelength was determined by scanning at the fluorescence wavelength. bEach emission was measured using excitation wavelengths. cFluorescence quantum yields were obtained by using an absolute PL quantum yield measurement system (C9920-1) of Hamamatsu Photonics.
The AIEE properties of products 3a,b, and 4a–e were evaluated in different EtOH/H2O (v/v) solvent mixtures (Table 3 and Figure S20 in Supporting Information File 1). The UV–vis spectra of all compounds in H2O are shown in Figure S17 (Supporting Information File 1). The fluorescence intensities of the ring-fused compounds 3a,b gradually decreased with increasing water fractions, and the ratio of water to ethanol Φ values (ΦH2O/ΦEtOH) was smaller than 1.0. These results indicated that aggregation was induced by π–π stacking interaction of the planar structures of compounds 3a and 3b in aqueous solution and that their excited states decayed by non-radiative pathways, resulting in ACQ. In contrast, the fluorescence intensities of compounds 4a–e increased in 100% water, and the ΦH2O/ΦEtOH value was higher than 1.0, indicating that AIEE occurred. In particular, the fluorescence of compounds 4a and 4e, bearing Br and CF3 groups, respectively, largely increased with the addition of H2O to an ethanolic solution (Figure 2) with ΦH2O/ΦEtOH values of 0.07 and 0.12, respectively, which were 7.0 and 15 times higher than those in ethanol (non-aggregated form). Because compounds 4a–e form an A–D–A system, charge disproportionation may affect intermolecular π–π interactions and lead to AIEE. The enhanced fluorescence was almost completely quenched by the addition of an 0.1 M HCl solution to solutions of compounds 4a and 4e (Figure 3). This result indicated that the protonation of the secondary amine disrupted the intermolecular π–π interactions or planarity of compounds 4 (Figure 4), and the rigid structure in solution was involved in AIEE.
Table 3: Fluorescence data for 3a,b, and 4a–e in H2O.
dissolved in H2O | ||||
compound | EXmax (nm)a | EMmax (nm)b | Φc | ΦH2O/ΦEtOHd |
3a | 468 | 559 | 0.01< | 1.0< |
3b | 355 | 550 | 0.01 | 0.5 |
4a | 413 | 486 | 0.07 | 7.0 |
4b | 431 | 553 | 0.02 | 2.0 |
4c | 358 | 467 | 0.01 | 1.0> |
4d | 421 | 460 | 0.01 | 1.0> |
4e | 415 | 452 | 0.12 | 15 |
aEach excitation wavelength was determined by scanning at the fluorescence wavelength. bEach emission was measured using excitation wavelengths. cFluorescence quantum yields were obtained by using an absolute PL quantum yield measurement system (C9920-1) of Hamamatsu Photonics. dThe ratio of Φ in H2O to Φ in EtOH.
![[1860-5397-18-60-2]](/bjoc/content/figures/1860-5397-18-60-2.png?scale=2.0&max-width=1024&background=FFFFFF)
Figure 2: Fluorescence spectral profiles of (a) 4a (10−5 M, Exmax = 413 nm) and (b) 4e (10−5 M, Exmax = 415 nm) in H2O/EtOH mixture with different water fractions.
Figure 2: Fluorescence spectral profiles of (a) 4a (10−5 M, Exmax = 413 nm) and (b) 4e (10−5 M, Exmax = 415 n...
![[1860-5397-18-60-3]](/bjoc/content/figures/1860-5397-18-60-3.png?scale=2.0&max-width=1024&background=FFFFFF)
Figure 3: Fluorescence spectral changes of (a) 4a (10−5 M, Exmax = 413 nm) and (b) 4e (10−5 M, Exmax = 415 nm) upon addition of 0.1 M HCl.
Figure 3: Fluorescence spectral changes of (a) 4a (10−5 M, Exmax = 413 nm) and (b) 4e (10−5 M, Exmax = 415 nm...
Figure 4: Protonation of N-methyl-4-((pyridin-2-yl)amino)-substituted maleimides 4 by 0.1 M HCl.
Figure 4: Protonation of N-methyl-4-((pyridin-2-yl)amino)-substituted maleimides 4 by 0.1 M HCl.
To compare the electronic natures of the compounds with and without AIEE, we performed TD-DFT calculations on each monomer of 3a, 4a, and 4e. The graphical representations of the highest occupied molecular orbital (HOMO) and the lowest unoccupied molecular orbital (LUMO) for the ground-state geometries of each monomer are shown in Figure 5. The dihedral angles between the pyrimidine and maleimide rings, the amine linkage, were nearly zero degrees for 4a and 4e in all three solvents, adopting a highly planar structure as well as compound 3a. The HOMO and LUMO were distributed on the entire structure of each compound owing to their high planarity in both the ground and excited states. The large overlap between these two frontier orbitals resulted in efficient absorption and emission.
![[1860-5397-18-60-5]](/bjoc/content/figures/1860-5397-18-60-5.png?scale=2.0&max-width=1024&background=FFFFFF)
Figure 5: Frontier molecular orbitals and HOMO–LUMO energy gaps of compounds 3a, 4a, and 4e for ground-state calculated by using the B3LYP/6-31G(d,p) level of theory in dichloromethane.
Figure 5: Frontier molecular orbitals and HOMO–LUMO energy gaps of compounds 3a, 4a, and 4e for ground-state ...
The calculated vertical excitation energies of compounds 3a, 4a, and 4e, along with their oscillator strengths, are listed in Table 4. The experimentally observed Exmax values for the S0→S1 transition are somewhat different from the calculated ones, but the order of Exmaxcalc corresponds to Exmaxobs other than in water, i.e. Exmax (4a) > Exmax (3a) > Exmax (4e) in dichloromethane and ethanol. As anticipated, the S0→S1 transition in both compounds is mostly dominated by charge transfer from the HOMO to the LUMO, corresponding to a π→π* electron transition. There was no significant change (≤7 nm) in the Exmax for compound 3a in the three solvents: Exmaxobs ranged from 468 to 471 nm and Exmaxcalc ranged from 464 to 468 nm. From calculations, a smaller solvent effect is seen in the Exmaxcalc values for compound 4a (484–488 nm) and 4e (404–407 nm), whereas the Exmaxobs were found at 476 nm in ethanol and 413 nm in water for 4a and at 378 nm in ethanol and 415 nm in water for compound 4e, respectively. This discrepancy between the calculated and observed Exmax values for compounds 4a and 4e suggests that aggregation occurs in water, and such behavior cannot be reproduced using the monomer model (non-aggregated form).
Table 4: Calculated excitation energies (E [Exmax]), oscillator strengths (f), and main components of the transition of the three lowest excited states for compounds 3a, 4a, and 4e using the TD-B3LYP/6-311+G(d,p)//B3LYP/6-31G(d,p) level of theory.
solvent | compound | excited states |
E
(eV) |
[Exmaxobs]a
(nm) |
[Exmaxcalc]
(nm) |
f |
main components of the transition
(% contribution) |
DCM | 3a | S1 | 2.67 | [471] | [464] | 0.224 | HOMO→LUMO (98) |
S2 | 3.56 | – | [348] | 0.121 | HOMO→LUMO+1 (97) | ||
S3 | 3.62 | – | [343] | 0 | HOMO-1→LUMO (94) | ||
4a | S1 | 2.54 | [477] | [488] | 0.386 | HOMO→LUMO (98) | |
S2 | 3.83 | – | [324] | 0 | HOMO-3→LUMO (62); HOMO-2→LUMO (36) | ||
S3 | 3.93 | – | [316] | 0.161 | HOMO-1→LUMO (94) | ||
4e | S1 | 3.07 | [373] | [404] | 0.308 | HOMO→LUMO (98) | |
S2 | 3.79 | – | [327] | 0 | HOMO-1→LUMO (50); HOMO-3→LUMO (47) | ||
S3 | 4.04 | – | [307] | 0.001 | HOMO-3→LUMO (50); HOMO-1→LUMO (48) | ||
EtOH | 3a | S1 | 2.66 | [468] | [466] | 0.239 | HOMO→LUMO (99) |
S2 | 3.57 | – | [348] | 0.140 | HOMO→LUMO+1 (98) | ||
S3 | 3.64 | – | [340] | 0 | HOMO-1→LUMO (94) | ||
4a | S1 | 2.55 | [476] | [486] | 0.409 | HOMO→LUMO (98) | |
S2 | 3.84 | – | [323] | 0 | HOMO-3→LUMO (60); HOMO-2→LUMO (37) | ||
S3 | 3.92 | – | [317] | 0.167 | HOMO-1→LUMO (94) | ||
4e | S1 | 3.05 | [378] | [406] | 0.329 | HOMO→LUMO (98) | |
S2 | 3.81 | – | [326] | 0 | HOMO-1→LUMO (51); HOMO-3→LUMO (46) | ||
S3 | 4.05 | – | [306] | 0.001 | HOMO-3→LUMO (51); HOMO-1→LUMO (47) | ||
H2O | 3a | S1 | 2.65 | [468] | [468] | 0.245 | HOMO→LUMO (99) |
S2 | 3.57 | – | [347] | 0.148 | HOMO→LUMO+1 (98) | ||
S3 | 3.65 | – | [339] | 0 | HOMO-1→LUMO (94) | ||
4a | S1 | 3.10 | [413] | [484] | 0.420 | HOMO→LUMO (98) | |
S2 | 3.85 | – | [322] | 0 | HOMO-3→LUMO (60); HOMO-2→LUMO (37) | ||
S3 | 3.92 | – | [317] | 0.169 | HOMO-1→LUMO (94) | ||
4e | S1 | 3.04 | [415] | [407] | 0.339 | HOMO→LUMO (98) | |
S2 | 3.81 | – | [325] | 0 | HOMO-1→LUMO (52); HOMO-3→LUMO (46) | ||
S3 | 4.05 | – | [306] | 0.001 | HOMO-3→LUMO (51); HOMO-1→LUMO (47) |
aExperimentally observed excitation wavelengths are listed in Table 2 and Table 3.
Conclusion
To discover low-molecular-weight AIEE-based luminogens, we synthesized pyrido[1,2-a]pyrrolo[3,4-d]pyrimidines 3a,b and N-methyl-4-((pyridin-2-yl)amino)-substituted maleimides 4a–e in moderately good yields by reacting functionalized maleimides with 2-aminopyridines under mild conditions. The presence of electron-donating or electron-withdrawing groups at position 5 of the 2-aminopyridine greatly affected the fluorescence properties of the products, and N-methyl-4-((pyridin-2-yl)amino)-substituted maleimides, containing electron-withdrawing groups formed an A–D–A system and exhibited AIEE properties in aqueous media. In particular, compounds 4a and 4e bearing electron-withdrawing groups (Br and CF3, respectively) exhibited a large fluorescence enhancement. A comparison of the calculated and observed Exmax using TD-DFT calculations revealed the basic features of compounds 4. Therefore, we envision N-methyl-4-((pyridin-2-yl)amino)-substituted maleimides based on an A–D–A system as novel luminogens for AIEE and are currently investigating the underlying mechanism and future biological applications.
Supporting Information
Supporting Information File 1: General information, synthesis of 3a,b, and 4a–e, experimental procedure of fluorescence, theoretical computation method measurements, NMR, UV–vis, and fluorescence spectra. | ||
Format: PDF | Size: 1.9 MB | Download |
References
-
Zhu, M.; Yang, C. Chem. Soc. Rev. 2013, 42, 4963–4976. doi:10.1039/c3cs35440g
Return to citation in text: [1] -
Wu, D.; Sedgwick, A. C.; Gunnlaugsson, T.; Akkaya, E. U.; Yoon, J.; James, T. D. Chem. Soc. Rev. 2017, 46, 7105–7123. doi:10.1039/c7cs00240h
Return to citation in text: [1] -
Staderini, M.; Aulić, S.; Bartolini, M.; Tran, H. N. A.; González-Ruiz, V.; Pérez, D. I.; Cabezas, N.; Martínez, A.; Martín, M. A.; Andrisano, V.; Legname, G.; Menéndez, J. C.; Bolognesi, M. L. ACS Med. Chem. Lett. 2013, 4, 225–229. doi:10.1021/ml3003605
Return to citation in text: [1] -
Iwahara, H.; Kushida, T.; Yamaguchi, S. Chem. Commun. 2016, 52, 1124–1127. doi:10.1039/c5cc08259e
Return to citation in text: [1] -
Hagimori, M.; Shigemitsu, Y.; Murakami, R.; Yokota, K.; Nishimura, Y.; Mizuyama, N.; Wang, B.-C.; Tai, C.-K.; Wang, S.-L.; Shih, T.-L.; Wu, K.-D.; Huang, Z.-S.; Tseng, S.-C.; Lu, J.-W.; Wei, H.-H.; Nagaoka, J.; Mukai, T.; Kawashima, S.; Kawashima, K.; Tominaga, Y. Dyes Pigm. 2016, 124, 196–202. doi:10.1016/j.dyepig.2015.09.017
Return to citation in text: [1] [2] [3] -
Ma, X.; Sun, R.; Cheng, J.; Liu, J.; Gou, F.; Xiang, H.; Zhou, X. J. Chem. Educ. 2016, 93, 345–350. doi:10.1021/acs.jchemed.5b00483
Return to citation in text: [1] [2] -
Chowdhury, S.; Rooj, B.; Dutta, A.; Mandal, U. J. Fluoresc. 2018, 28, 999–1021. doi:10.1007/s10895-018-2263-y
Return to citation in text: [1] [2] -
Pramanik, S.; Bhalla, V.; Kim, H. M.; Singh, H.; Lee, H. W.; Kumar, M. Chem. Commun. 2015, 51, 15570–15573. doi:10.1039/c5cc05406k
Return to citation in text: [1] [2] -
Shan, Y.; Yao, W.; Liang, Z.; Zhu, L.; Yang, S.; Ruan, Z. Dyes Pigm. 2018, 156, 1–7. doi:10.1016/j.dyepig.2018.03.060
Return to citation in text: [1] [2] -
Wang, H.; Zhao, E.; Lam, J. W. Y.; Tang, B. Z. Mater. Today 2015, 18, 365–377. doi:10.1016/j.mattod.2015.03.004
Return to citation in text: [1] [2] [3] [4] -
Bull, J. A.; Mousseau, J. J.; Pelletier, G.; Charette, A. B. Chem. Rev. 2012, 112, 2642–2713. doi:10.1021/cr200251d
Return to citation in text: [1] -
Baumann, M.; Baxendale, I. R. Beilstein J. Org. Chem. 2013, 9, 2265–2319. doi:10.3762/bjoc.9.265
Return to citation in text: [1] -
Kon, A.; Takagaki, K.; Kawasaki, H.; Nakamura, T.; Endo, M. J. Biochem. 1991, 110, 132–135. doi:10.1093/oxfordjournals.jbchem.a123531
Return to citation in text: [1] -
Domljanovic, I.; Rexen Ulven, E.; Ulven, T.; Thomsen, R. P.; Okholm, A. H.; Kjems, J.; Voss, A.; Taskova, M.; Astakhova, K. ACS Omega 2018, 3, 7580–7586. doi:10.1021/acsomega.8b00424
Return to citation in text: [1] -
Velázquez-Olvera, S.; Salgado-Zamora, H.; Velázquez-Ponce, M.; Campos-Aldrete, E.; Reyes-Arellano, A.; Pérez-González, C. Chem. Cent. J. 2012, 6, 83. doi:10.1186/1752-153x-6-83
Return to citation in text: [1] -
Hagimori, M.; Nishimura, Y.; Mizuyama, N.; Shigemitsu, Y. Dyes Pigm. 2019, 171, 107705. doi:10.1016/j.dyepig.2019.107705
Return to citation in text: [1] -
Hagimori, M.; Temma, T.; Mizuyama, N.; Uto, T.; Yamaguchi, Y.; Tominaga, Y.; Mukai, T.; Saji, H. Sens. Actuators, B 2015, 213, 45–52. doi:10.1016/j.snb.2015.02.063
Return to citation in text: [1] -
Hagimori, M.; Matsui, S.; Mizuyama, N.; Yokota, K.; Nagaoka, J.; Tominaga, Y. Eur. J. Org. Chem. 2009, 5847–5853. doi:10.1002/ejoc.200900783
Return to citation in text: [1] -
Hagimori, M.; Mizuyama, N.; Hisadome, Y.; Nagaoka, J.; Ueda, K.; Tominaga, Y. Tetrahedron 2007, 63, 2511–2518. doi:10.1016/j.tet.2006.12.031
Return to citation in text: [1] -
Yang, W.; Li, C.; Zhang, M.; Zhou, W.; Xue, R.; Liu, H.; Li, Y. Phys. Chem. Chem. Phys. 2016, 18, 28052–28060. doi:10.1039/c6cp04755f
Return to citation in text: [1] -
Ding, D.; Li, K.; Liu, B.; Tang, B. Z. Acc. Chem. Res. 2013, 46, 2441–2453. doi:10.1021/ar3003464
Return to citation in text: [1] -
Hu, R.; Lager, E.; Aguilar-Aguilar, A.; Liu, J.; Lam, J. W. Y.; Sung, H. H. Y.; Williams, I. D.; Zhong, Y.; Wong, K. S.; Peña-Cabrera, E.; Tang, B. Z. J. Phys. Chem. C 2009, 113, 15845–15853. doi:10.1021/jp902962h
Return to citation in text: [1] -
Shigemitsu, Y.; Komiya, K.; Mizuyama, N.; Hagimori, M.; Tominaga, Y. Res. Lett. Org. Chem. 2009, 413219. doi:10.1155/2009/413219
Return to citation in text: [1] -
Hagimori, M.; Mizuyama, N.; Yokota, K.; Morinaga, O.; Yamaguchi, Y.; Saji, H.; Tominaga, Y. Heterocycles 2011, 83, 1983–1988. doi:10.3987/com-11-12285
Return to citation in text: [1] -
Kizaki, K.; Imoto, H.; Kato, T.; Naka, K. Tetrahedron 2015, 71, 643–647. doi:10.1016/j.tet.2014.12.023
Return to citation in text: [1]
1. | Zhu, M.; Yang, C. Chem. Soc. Rev. 2013, 42, 4963–4976. doi:10.1039/c3cs35440g |
2. | Wu, D.; Sedgwick, A. C.; Gunnlaugsson, T.; Akkaya, E. U.; Yoon, J.; James, T. D. Chem. Soc. Rev. 2017, 46, 7105–7123. doi:10.1039/c7cs00240h |
3. | Staderini, M.; Aulić, S.; Bartolini, M.; Tran, H. N. A.; González-Ruiz, V.; Pérez, D. I.; Cabezas, N.; Martínez, A.; Martín, M. A.; Andrisano, V.; Legname, G.; Menéndez, J. C.; Bolognesi, M. L. ACS Med. Chem. Lett. 2013, 4, 225–229. doi:10.1021/ml3003605 |
7. | Chowdhury, S.; Rooj, B.; Dutta, A.; Mandal, U. J. Fluoresc. 2018, 28, 999–1021. doi:10.1007/s10895-018-2263-y |
8. | Pramanik, S.; Bhalla, V.; Kim, H. M.; Singh, H.; Lee, H. W.; Kumar, M. Chem. Commun. 2015, 51, 15570–15573. doi:10.1039/c5cc05406k |
9. | Shan, Y.; Yao, W.; Liang, Z.; Zhu, L.; Yang, S.; Ruan, Z. Dyes Pigm. 2018, 156, 1–7. doi:10.1016/j.dyepig.2018.03.060 |
10. | Wang, H.; Zhao, E.; Lam, J. W. Y.; Tang, B. Z. Mater. Today 2015, 18, 365–377. doi:10.1016/j.mattod.2015.03.004 |
7. | Chowdhury, S.; Rooj, B.; Dutta, A.; Mandal, U. J. Fluoresc. 2018, 28, 999–1021. doi:10.1007/s10895-018-2263-y |
8. | Pramanik, S.; Bhalla, V.; Kim, H. M.; Singh, H.; Lee, H. W.; Kumar, M. Chem. Commun. 2015, 51, 15570–15573. doi:10.1039/c5cc05406k |
9. | Shan, Y.; Yao, W.; Liang, Z.; Zhu, L.; Yang, S.; Ruan, Z. Dyes Pigm. 2018, 156, 1–7. doi:10.1016/j.dyepig.2018.03.060 |
10. | Wang, H.; Zhao, E.; Lam, J. W. Y.; Tang, B. Z. Mater. Today 2015, 18, 365–377. doi:10.1016/j.mattod.2015.03.004 |
5. | Hagimori, M.; Shigemitsu, Y.; Murakami, R.; Yokota, K.; Nishimura, Y.; Mizuyama, N.; Wang, B.-C.; Tai, C.-K.; Wang, S.-L.; Shih, T.-L.; Wu, K.-D.; Huang, Z.-S.; Tseng, S.-C.; Lu, J.-W.; Wei, H.-H.; Nagaoka, J.; Mukai, T.; Kawashima, S.; Kawashima, K.; Tominaga, Y. Dyes Pigm. 2016, 124, 196–202. doi:10.1016/j.dyepig.2015.09.017 |
6. | Ma, X.; Sun, R.; Cheng, J.; Liu, J.; Gou, F.; Xiang, H.; Zhou, X. J. Chem. Educ. 2016, 93, 345–350. doi:10.1021/acs.jchemed.5b00483 |
25. | Kizaki, K.; Imoto, H.; Kato, T.; Naka, K. Tetrahedron 2015, 71, 643–647. doi:10.1016/j.tet.2014.12.023 |
4. | Iwahara, H.; Kushida, T.; Yamaguchi, S. Chem. Commun. 2016, 52, 1124–1127. doi:10.1039/c5cc08259e |
5. | Hagimori, M.; Shigemitsu, Y.; Murakami, R.; Yokota, K.; Nishimura, Y.; Mizuyama, N.; Wang, B.-C.; Tai, C.-K.; Wang, S.-L.; Shih, T.-L.; Wu, K.-D.; Huang, Z.-S.; Tseng, S.-C.; Lu, J.-W.; Wei, H.-H.; Nagaoka, J.; Mukai, T.; Kawashima, S.; Kawashima, K.; Tominaga, Y. Dyes Pigm. 2016, 124, 196–202. doi:10.1016/j.dyepig.2015.09.017 |
10. | Wang, H.; Zhao, E.; Lam, J. W. Y.; Tang, B. Z. Mater. Today 2015, 18, 365–377. doi:10.1016/j.mattod.2015.03.004 |
20. | Yang, W.; Li, C.; Zhang, M.; Zhou, W.; Xue, R.; Liu, H.; Li, Y. Phys. Chem. Chem. Phys. 2016, 18, 28052–28060. doi:10.1039/c6cp04755f |
21. | Ding, D.; Li, K.; Liu, B.; Tang, B. Z. Acc. Chem. Res. 2013, 46, 2441–2453. doi:10.1021/ar3003464 |
22. | Hu, R.; Lager, E.; Aguilar-Aguilar, A.; Liu, J.; Lam, J. W. Y.; Sung, H. H. Y.; Williams, I. D.; Zhong, Y.; Wong, K. S.; Peña-Cabrera, E.; Tang, B. Z. J. Phys. Chem. C 2009, 113, 15845–15853. doi:10.1021/jp902962h |
16. | Hagimori, M.; Nishimura, Y.; Mizuyama, N.; Shigemitsu, Y. Dyes Pigm. 2019, 171, 107705. doi:10.1016/j.dyepig.2019.107705 |
17. | Hagimori, M.; Temma, T.; Mizuyama, N.; Uto, T.; Yamaguchi, Y.; Tominaga, Y.; Mukai, T.; Saji, H. Sens. Actuators, B 2015, 213, 45–52. doi:10.1016/j.snb.2015.02.063 |
18. | Hagimori, M.; Matsui, S.; Mizuyama, N.; Yokota, K.; Nagaoka, J.; Tominaga, Y. Eur. J. Org. Chem. 2009, 5847–5853. doi:10.1002/ejoc.200900783 |
19. | Hagimori, M.; Mizuyama, N.; Hisadome, Y.; Nagaoka, J.; Ueda, K.; Tominaga, Y. Tetrahedron 2007, 63, 2511–2518. doi:10.1016/j.tet.2006.12.031 |
23. | Shigemitsu, Y.; Komiya, K.; Mizuyama, N.; Hagimori, M.; Tominaga, Y. Res. Lett. Org. Chem. 2009, 413219. doi:10.1155/2009/413219 |
24. | Hagimori, M.; Mizuyama, N.; Yokota, K.; Morinaga, O.; Yamaguchi, Y.; Saji, H.; Tominaga, Y. Heterocycles 2011, 83, 1983–1988. doi:10.3987/com-11-12285 |
13. | Kon, A.; Takagaki, K.; Kawasaki, H.; Nakamura, T.; Endo, M. J. Biochem. 1991, 110, 132–135. doi:10.1093/oxfordjournals.jbchem.a123531 |
14. | Domljanovic, I.; Rexen Ulven, E.; Ulven, T.; Thomsen, R. P.; Okholm, A. H.; Kjems, J.; Voss, A.; Taskova, M.; Astakhova, K. ACS Omega 2018, 3, 7580–7586. doi:10.1021/acsomega.8b00424 |
15. | Velázquez-Olvera, S.; Salgado-Zamora, H.; Velázquez-Ponce, M.; Campos-Aldrete, E.; Reyes-Arellano, A.; Pérez-González, C. Chem. Cent. J. 2012, 6, 83. doi:10.1186/1752-153x-6-83 |
11. | Bull, J. A.; Mousseau, J. J.; Pelletier, G.; Charette, A. B. Chem. Rev. 2012, 112, 2642–2713. doi:10.1021/cr200251d |
12. | Baumann, M.; Baxendale, I. R. Beilstein J. Org. Chem. 2013, 9, 2265–2319. doi:10.3762/bjoc.9.265 |
5. | Hagimori, M.; Shigemitsu, Y.; Murakami, R.; Yokota, K.; Nishimura, Y.; Mizuyama, N.; Wang, B.-C.; Tai, C.-K.; Wang, S.-L.; Shih, T.-L.; Wu, K.-D.; Huang, Z.-S.; Tseng, S.-C.; Lu, J.-W.; Wei, H.-H.; Nagaoka, J.; Mukai, T.; Kawashima, S.; Kawashima, K.; Tominaga, Y. Dyes Pigm. 2016, 124, 196–202. doi:10.1016/j.dyepig.2015.09.017 |
6. | Ma, X.; Sun, R.; Cheng, J.; Liu, J.; Gou, F.; Xiang, H.; Zhou, X. J. Chem. Educ. 2016, 93, 345–350. doi:10.1021/acs.jchemed.5b00483 |
10. | Wang, H.; Zhao, E.; Lam, J. W. Y.; Tang, B. Z. Mater. Today 2015, 18, 365–377. doi:10.1016/j.mattod.2015.03.004 |
© 2022 Hagimori et al.; licensee Beilstein-Institut.
This is an open access article licensed under the terms of the Beilstein-Institut Open Access License Agreement (https://www.beilstein-journals.org/bjoc/terms), which is identical to the Creative Commons Attribution 4.0 International License (https://creativecommons.org/licenses/by/4.0). The reuse of material under this license requires that the author(s), source and license are credited. Third-party material in this article could be subject to other licenses (typically indicated in the credit line), and in this case, users are required to obtain permission from the license holder to reuse the material.