Abstract
Organic–inorganic magnetic hybrid materials (MHMs) combine a nonmagnetic and a magnetic component by means of electrostatic interactions or covalent bonds, and notable features can be achieved. Herein, we describe an application of a self-assembled material based on ferrite associated with β-cyclodextrin (Fe-Ni/Zn/βCD) at the nanoscale level. This MHM and pure ferrite (Fe-Ni/Zn) were used as an adsorbent system for Cr3+ and Cr2O72− ions in aqueous solutions. Prior to the adsorption studies, both ferrites were characterized in order to determine the particle size distribution, morphology and available binding sites on the surface of the materials. Microscopy analysis demonstrated that both ferrites present two different size domains, at the micro- and nanoscale level, with the latter being able to self-assemble into larger particles. Fe-Ni/Zn/βCD presented smaller particles and a more homogeneous particle size distribution. Higher porosity for this MHM compared to Fe-Ni/Zn was observed by Brunauer–Emmett–Teller isotherms and positron-annihilation-lifetime spectroscopy. Based on the pKa values, potentiometric titrations demonstrated the presence of βCD in the inorganic matrix, indicating that the lamellar structures verified by transmission electronic microscopy can be associated with βCD assembled structures. Colloidal stability was inferred as a function of time at different pH values, indicating the sedimentation rate as a function of pH. Zeta potential measurements identified an amphoteric behavior for the Fe-Ni/Zn/βCD, suggesting its better capability to remove ions (cations and anions) from aqueous solutions compared to that of Fe-Ni/Zn.
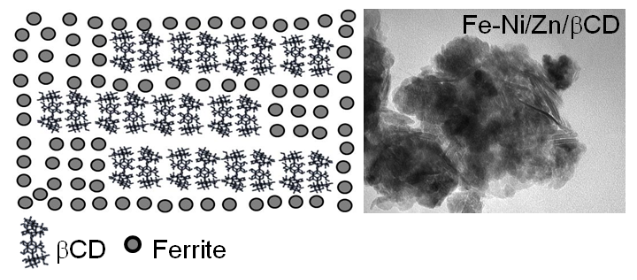
Graphical Abstract
Introduction
Organic–inorganic hybrid materials (HMs) are often prepared by assembling organic and inorganic molecules based on electrostatic interactions or chemical bonding between them, which will leads to an unpredictable stoichiometry [1]. The structures and properties of HMs depend on the nature of both components, organic and inorganic, and also on the synthesis process, which can be carried out by metal or organic hydrolyses [2,3]. Controlling the method of HM synthesis could lead to a predictable crystal structure and homogeneous particle size distribution [4]. Additionally, surface properties could be modulated by selecting an appropriate organic molecule with desirable functional groups in its structure. In particular, magnetic organic–inorganic hybrid materials (MHMs) have attracted considerable attention based on their multifunctional and biocompatible properties [2,5,6]. Moreover, MHMs can present a greater number of applications than nonmagnetic hybrid materials when their suspensions are used.
These MHMs systems are susceptible to external magnetic fields because of the strong magnetic interactions existing among the magnetized particles, which are able to modify the colloidal and rheological properties of the solutions. Thus, functions based on their magnetic characteristics such as conduction and accumulation in a system under an external magnetic field can be realized. In this sense, colloidal systems of MHMs have shown an increasing number of applications in many different technologies, including ferrofluids [7], magnetic separators [8], magnetic resonance imaging [9], hyperthermia [10], and water treatment [4].
Nickel-zinc ferrites (Fe-Ni/Zn) are one of these versatile magnetic materials, since these systems present high magnetic saturation, Curie temperature and chemical stability. Additionally, low coercivity and biodegradability have been observed in Fe-Ni/Zn, being an interesting part of the inorganic component in the MHM structure [11]. The organic molecule in the MHM should present specific characteristics in order to improve the material application, including available binding sites, to bond or interact through intermolecular forces with the inorganic matrix, and high surface area, which is important to improve the MHM adsorption and adhesion properties. In order to design a MHM based on Fe-Ni/Zn with adsorption properties for environmental use, cyclodextrins (CDs) can be associated with the inorganic matrix as an interesting strategy, since these macromolecules have been used for several devices with different properties, from light-responsive matrices to molecular recognition materials [12,13].
CDs are oligosaccharides commonly formed by six, seven or eight α(1→4) linked-D-glucopyranoside units, named αCD, βCD and γCD, respectively. These macromolecules have a rigid and well-defined structure with a toroidal shape, in which a variety of organic and inorganic guest molecules can be inserted into their cavities, resulting in the formation of inclusion complexes [14-17]. Beyond these characteristics, it has been reported in the literature that CDs self-assemble into large aggregates [18-20], suggesting their uses as size-modulator molecules [21], similar to other amphiphilic molecules [22-25]. Based on these interesting properties for both systems, CDs and Fe-Ni/Zn can be used to synthesize a MHM with large number of applications. Moreover, due to the arrangement of CDs, their primary and secondary hydroxy groups may be able to interact with a ferrite structure, through covalent bonds or also by intramolecular interactions. These hydroxy groups could improve the adsorption properties of the MHM by including guest molecules in CD cavities or also by allowing the assembly process. Recently, adsorption materials have become one of the most versatile and widely used technologies to remove heavy metals from industrial wastewater, including chrome in its different oxidation states [4,26]. Although activated carbon has been frequently applied for this purpose, magnetic adsorbent materials have also demonstrated their potential applicability in the past few years, because of their easy separation properties.
Herein, Fe-Ni/Zn and the MHM prepared by using Fe-Ni/Zn and βCD (Fe-Ni/Zn/βCD) were synthesized by adapting a method previously described in the literature [7]. These magnetic materials were characterized in the solid state by X-ray powder diffraction (XRD), Fourier transform infrared spectroscopy (FTIR), and thermal analysis (TG/DTA), and by their magnetic behavior in aqueous suspension (see Supporting Information File 1). Fe-Ni/Zn and Fe-Ni/Zn/βCD nanoparticles morphologies were investigated in the solid state by scanning electronic microscopy (SEM), transmission electronic microscopy (TEM), and atomic force microscopy (AFM). Size distribution and colloidal suspension stability were characterized by dynamic light scattering (DLS) and sedimentation kinetic studies by using UV–vis spectroscopy. Ferrite binding sites were characterized by zeta potential (ZP) and potentiometric titration. The free volume for both materials was evaluated by Brunauer–Emmett–Teller isotherm (BET) and positron-annihilation-lifetime spectroscopy (PALS). Thus, considering the advanced functional properties achieved by βCD insertion in the inorganic matrix identified by the above experiments and the MHM capability to self-assemble, the adsorption properties for both ferrites using chrome ions (Cr3+ and Cr2O72−) in aqueous solutions were tested and evaluated by ZP measurements.
Results and Discussion
Fe-Ni/Zn and Fe-Ni/Zn/βCD size characterization
In order to investigate the Fe-Ni/Zn and Fe-Ni/Zn/βCD morphology and size distribution at different aggregation levels, these materials were investigated in the solid state by SEM, TEM and AFM and also in aqueous suspension by using DLS. AFM images of Fe-Ni/Zn and Fe-Ni/Zn/βCD are presented in Figure 1. Based on these images, nanoparticles below 100 nm were verified for these materials. Looking closer, Fe-Ni/Zn/βCD presents smaller nanoparticles in a range of 20 to 50 nm, while the Fe-Ni/Zn consisted of domains higher than 50 nm. The smaller particles observed for the Fe-Ni/Zn/βCD particles could be due to the size-modulator effect of CDs [19,20,27,28], which could act as a nanoreactor for ferrite synthesis, preventing the particles growing during the ferrite synthesis (nucleation process).
![[1860-5397-8-215-1]](/bjoc/content/figures/1860-5397-8-215-1.png?scale=2.0&max-width=1024&background=FFFFFF)
Figure 1: AFM images of (a) Fe-Ni/Zn and (b) Fe-Ni/Zn/βCD.
Figure 1: AFM images of (a) Fe-Ni/Zn and (b) Fe-Ni/Zn/βCD.
In order to gain insight into the microstructure of these assembled materials, SEM and TEM were also carried out. TEM images of the magnetic materials, demonstrating their structure from the nanoscale level up to the aggregate particles, are shown in Figure 2a and Figure 2b. In addition, the TEM image for the Fe-Ni/Zn/βCD showed aligned structures in the nanoparticle matrix, which can correspond to the assembled βCD structures in the MHM. These lamellar self-assembled structures are similar to the βCD crystals described previously in the literature [29], confirming the presence of the macromolecule in the ferrite nanoparticles matrix. SEM images, Figure 2c and Figure 2d, demonstrate larger assembled particles (above 500 nm). It is interesting to note that when βCD is used to prepare the ferrite nanoparticles, size domains are smaller than those observed for the material prepared without the macromolecule. These results corroborate the hypothesis considering the size-modulator effect of βCD proposed previously based on the analysis of the AFM images.
![[1860-5397-8-215-2]](/bjoc/content/figures/1860-5397-8-215-2.png?scale=2.0&max-width=1024&background=FFFFFF)
Figure 2: TEM images of (a) Fe-Ni/Zn and (b) Fe-Ni/Zn/βCD and SEM images of (c) Fe-Ni/Zn and (d) Fe-Ni/Zn/βCD.
Figure 2: TEM images of (a) Fe-Ni/Zn and (b) Fe-Ni/Zn/βCD and SEM images of (c) Fe-Ni/Zn and (d) Fe-Ni/Zn/βCD....
Fe-Ni/Zn and Fe-Ni/Zn/βCD were also analyzed in aqueous suspensions, at pH 7, (Figure 3). The ferrite aggregation process was confirmed, Figure 3a and Figure 3b, in which particle size distributions of about 2.8 and 2.3 μm were observed for Fe-Ni/Zn and Fe-Ni/Zn/βCD, respectively. In the presence of βCD a more homogeneous size distribution was verified, suggesting that βCD is able to minimize the ferrite coalescence process in aqueous solution. The ferrite aggregation process may occur in low zeta potential values (−10 mV, see zeta potential data) and is insufficient to avoid van der Waals attraction [30]; however, at pH 7 this self-assembly behavior can also be verified. Evidence for the ferrite aggregation process is observed in aqueous suspensions when these materials are sonicated, Figures 3c and Figure 3d, in which the assembled structures are disrupted in order to obtain the nanoparticles. Once again, Fe-Ni/Zn/βCD presented a smaller particles distribution (average size of about 85 nm) with a more homogenous size distribution than that prepared without βCD (Fe-Ni/Zn about 150 nm). These results are in accordance with those verified by AFM and TEM, in which Fe-Ni/Zn/βCD presented smaller nanoparticles than pure ferrite in the solid state. Although some discrepancy between the DLS and AFM particle size distributions has been observed, it has been previously reported in the literature for other systems [31,32].
![[1860-5397-8-215-3]](/bjoc/content/figures/1860-5397-8-215-3.png?scale=2.0&max-width=1024&background=FFFFFF)
Figure 3: DLS measurements for before (a) and (b) and after (c) and (d) the sonication process.
Figure 3: DLS measurements for before (a) and (b) and after (c) and (d) the sonication process.
Textural characterization by gas adsorption
BET isotherms were carried out to investigate the free volume of the ferrites. Fe-Ni/Zn was able to adsorb gas at 115 cm3 g−1, showing a type IV isotherm by (Brunauer, Deming, Deming, and Teller) BDDT classification [33], which is characteristic of mesoporous materials, and its hysteresis loop closed at p/p0 = 0.2. Adsorbing capacity for the Fe-Ni/Zn/βCD was 125 cm3 of gas per gram, presenting an intermediary isotherm characteristic that changes from meso- to microporosity, and its hysteresis loop closed at p/p0 = 0.4, demonstrating that the presence of βCD implies a minor difficulty in adsorption.
Textural characteristics for the ferrite (Fe-Ni/Zn), which has a specific surface area of 127 m2 g−1, changed substantially with the inclusion of βCD in the inorganic matrix, increasing the specific surface area to 191 m2 g−1 (an increase of 50.4%). This surface area variation is mainly due to the microporosity increasing by the inclusion of the βCD in the inorganic matrix. Fe-Ni/Zn presented a fractal dimension (D) of 2.772, which increased to 2.934 with the macromolecule insertion, showing a significant growth in the surface roughness, since the D value of a surface with maximum roughness is 3. This result is in accordance with microscopy analysis in the solid state, which shows different morphologies for these two samples.
Determination of free volume by PALS
The free volume was measured by positron probe through the PALS technique, where the longest component of the positron-lifetime spectrum, τ3 (the o-Ps pick-off lifetime), can be correlated with free volume holes in condensed matter. According to this model, τ3 grows leading to an increasing in the free volume radius, based on a spherical-cavity model [34,35]. The calculated free volume dimension has values of Vf = 69.9 Å3 and Vf = 115.9 Å3 for Fe-Ni/Zn and Fe-Ni/Zn/βCD, respectively; corresponding to an increase of 65% of the free volume (ΔVf = 46 Å3). These results corroborate BET isotherm studies, which pointed out a greater porosity in the material prepared by using βCD.
Potentiometric titrations
Potentiometric titrations were recorded in order to determine the number of ionizable sites for the magnetic materials through the first derivative of the titration curve. As can be seen in Figure 4a and Figure 4b, the first derivative of the titration curves pointed out several transitions, demonstrating that ferrite could be considered a polyprotic acid, probably due to the ionization of the different groups on the ferrite surfaces. It can also be observed that the potentiometric curve for Fe-Ni/Zn/βCD exhibits at least four transitions, two in addition to the pure Fe-Ni/Zn. The pKa values of the ferrites were calculated by using the Henderson–Hasselbalch Equation 1, at the point where [A−] = [HA] [36]:
![[1860-5397-8-215-4]](/bjoc/content/figures/1860-5397-8-215-4.png?scale=2.0&max-width=1024&background=FFFFFF)
Figure 4: Potentiometric curves of the (a) Fe-Ni/Zn and (b) Fe-Ni/Zn/βCD.
Figure 4: Potentiometric curves of the (a) Fe-Ni/Zn and (b) Fe-Ni/Zn/βCD.
Transitions at pKa 6.5 and 13.5 were attributed to the ionization of ferrite groups in the MHM system, since Fe-Ni/Zn also presented close transition values (pKa 6.5 and 12.6). Thus, transitions at pKa 5.5 and 9.4 in the Fe-Ni/Zn/βCD were attributed to the ionization of βCD primary and secondary hydroxy groups. These results emphasize that βCD is part of the inorganic matrix, corroborating the data obtained by TEM, in which lamellar assembled structures were observed in the MHM matrix.
Zeta potential
Particles in contact with an aqueous electrolyte solution acquire a surface charge as a result of adsorption or ionization processes. In order to evaluate the electrostatic repulsion between dispersed ferrite particles in solution, the electrical surface potential was evaluated by using ZP, computed from electrophoretic mobility measurements [37]. Figure 5 depicts the ZP variation as a function of the pH for the aqueous suspension of the ferrites, in which the solutions were tuned by using nitric acid or sodium hydroxide. These curves demonstrated that the zeta potential values are negative under the studied experimental conditions, and a pH dependency was also verified, once the ZP values become more negative increasing the pH of the aqueous suspensions.
![[1860-5397-8-215-5]](/bjoc/content/figures/1860-5397-8-215-5.png?scale=2.0&max-width=1024&background=FFFFFF)
Figure 5: Zeta potential curves as a function of pH for the (○) Fe-Ni/Zn and (▲) Fe-Ni/Zn/βCD.
Figure 5: Zeta potential curves as a function of pH for the (○) Fe-Ni/Zn and (▲) Fe-Ni/Zn/βCD.
The negative values in the pH range could be attributed to (i) ionization of R–OH groups after pH 6.5, which causes the formation of negative charges on the ferrite surface [38], and/or (ii) preferential adsorption of nitrate anions on the particle surface before this pH, since ferrites can become less hydrophilic upon protonation, and it is known that NO3− exhibits a preferential adsorption on weakly hydrated surfaces [39]. The isoelectric point (IP) of ferrites is close to pH 7 [40], thus inflexions observed close to this value for both systems may be an apparent IP, which was masked by competition between the ionization of Fe–OH groups and preferential NO3− adsorption. In other materials, negative ZP values have been also found in the pH range scanned from 1 to 13 [41-43].
Although the potentiometric titration demonstrated that ferrites present different transitions due to the different ionization sites, ZP titrations did not show the same behavior. A reasonable explanation for this phenomenon is based on the sensitivity of these analytical techniques. Potentiometry measures the electrical potential difference established through the electrode membrane, being directly dependent on the activity of H3O+ ions in the bulk solution, since its rate of diffusion is proportional to the concentration. ZP measurements obtained by Doppler electrophoresis are dependent on the Brownian diffusion of the particles in an electric field, which depends on the surface charge and size, and the size and charge polydispersities, as well as the aggregation state, among other phenomena; which are hard to control in a suspension material.
Sedimentation studies
Information about the colloidal stability of these ferrite suspensions can be inferred from the optical obscuration changes of the optic path by visible light at 700 nm as a function of time [40]. Figure 6 shows the obscuration (Ob) relative to its absorbance at time t = 0 s (Ob,0), plotted as a function of time for different pH values. Although the overall tendency of Ob/Ob,0 is to decrease over time due to particle sedimentation, the results obtained at different pH do not overlap, indicating that there is a clear pH dependence on the sedimentation rate. This pH dependence corresponds to the charge variation on the ferrite surface, which was also observed in the ZP titrations.
![[1860-5397-8-215-6]](/bjoc/content/figures/1860-5397-8-215-6.png?scale=2.0&max-width=1024&background=FFFFFF)
Figure 6: Relative optical obscuration (Ob/Ob,0) as a function of time for (a) Fe-Ni/Zn and (b) Fe-Ni/Zn/βCD.
Figure 6: Relative optical obscuration (Ob/Ob,0) as a function of time for (a) Fe-Ni/Zn and (b) Fe-Ni/Zn/βCD.
Since light scattering and absorption properties upon sedimentation are complicated phenomena, only the initial slopes, S = d(Ob/Ob,0)t→0/dt, of the obscuration curves were used in order to evaluate the sedimentation rate, as suggested by Plaza et al. [40]. Figure 7 shows the initial sedimentation rate for both magnetic systems. It can be observed that S values are greater in magnitude for the Fe-Ni/Zn/βCD than for the Fe-Ni/Zn: once at neutral pH the initial sedimentation is faster. The maximum absolute S (i.e., |S|) can be related to the absence of electrostatic repulsion between the particles in the pH vicinity of the apparent IP, and particle aggregation could occur under these conditions. This is a direct consequence of the greater number of hydroxy groups in the Fe-Ni/Zn/βCD system.
![[1860-5397-8-215-7]](/bjoc/content/figures/1860-5397-8-215-7.png?scale=2.0&max-width=1024&background=FFFFFF)
Figure 7: Obscuration curves for the Fe-Ni/Zn (○) and Fe-Ni/Zn/βCD (▲) as a function of pH.
Figure 7: Obscuration curves for the Fe-Ni/Zn (○) and Fe-Ni/Zn/βCD (▲) as a function of pH.
At pH ≈ 1, both ferrites have approximately the same and slow rate of sedimentation, probably due to NO3– adsorption. However, at pH ≈ 13, |S| is smaller for the Fe-Ni/Zn/βCD than for pure ferrite; in spite of the fact that the ZP results demonstrated the same values for both materials. The smaller particles and the greater presence of hydroxy groups of the Fe-Ni/Zn/βCD could be responsible for the slower sedimentation rate at this pH value.
Adsorption studies
Adsorption studies were carried out in order to compare the ion adsorption capacity of these magnetic materials in aqueous solution. ZP measurements were used to gain insights into the adsorption processes of Cr3+ and Cr2O72− ions. Figure 8 shows how the ZP values change as a function of the ion concentration. The ZP titration curves indicated that the MHM is able to adsorb both ions (Cr3+ and Cr2O72−) on its surface, since the ZP values (|ZP|) increase in their presence. These data suggest an amphoteric characteristic of Fe-Ni/Zn/βCD, which can interact with positive and negative species through ion–dipole or ion–ion interactions. Changes in the ZP values have been observed in the literature for different ferrites after the ion-adsorption process, indicating the affinity between them [44].
![[1860-5397-8-215-8]](/bjoc/content/figures/1860-5397-8-215-8.png?scale=2.0&max-width=1024&background=FFFFFF)
Figure 8: Adsorption curves of (a) Cr3+ and (b) Cr2O72− ions using Fe-Ni/Zn and Fe-Ni/Zn/βCD aqueous suspensions.
Figure 8: Adsorption curves of (a) Cr3+ and (b) Cr2O72− ions using Fe-Ni/Zn and Fe-Ni/Zn/βCD aqueous suspensi...
Comparing qualitatively the interaction involving the MHM with both ions, it is possible to observe its greater affinity for the Cr2O72− than the Cr3+, since for the latter a constant ZP value was observed above 0.4 mmol L−1, while a plateau was not reached for the Cr2O72− titration. This result also demonstrated that the MHM are able to adsorb more than 10 mmol of this anion per liter (maximum concentration tested), which is greater than that of approximately 0.4 mmol L−1 for the Cr3+ cation. The MHM capacity to adsorb Cr3+ ions (about 19 mg g−1 of MHM) was higher than that observed for MnFe2O4 ferrite (7.6 mg g−1 of MnFe2O4) to remove Cr6+ from aqueous solutions, described previously [4]. Thus, the inclusion of βCD in the inorganic matrix increased the ion adsorption, demonstrating the importance of choosing the organic component in a hybrid material carefully. Fe-Ni/Zn adsorption capacity for the Cr3+ cation was similar to that observed when βCD was used in the MHM. However without βCD, Fe-Ni/Zn was not able to adsorb the Cr2O72−, since no change in the ZP values was verified, indicating that Fe-Ni/Zn and the anion did not interact in aqueous suspension.
Conclusion
Hybrid magnetic nanoparticles based on ferrite and βCD were prepared and characterized in the solid state and in aqueous suspension, and their properties were compared with pure ferrite. Structural analysis pointed out that the synthesis approach was able to incorporate βCD in the ferrite matrix, which allowed us to keep the important βCD characteristics in the final magnetic material. Ferrite morphology in the solid state was affected by βCD insertion, probably due to its size and key role as modulator during inorganic nucleation. Ferrites presented at least three different organization scales, from the micrometric to the nanometric level, and on the nanometric scale it was possible to verify the material organization and particle size distribution. Lower domains, and greater specific area and free volume were observed for the Fe-Ni/Zn/βCD material. On the micrometric level, both ferrites have comparable behavior, with quite similar size distribution and ZP values, although Fe-Ni/Zn/βCD presents a slower sedimentation at high pH values. Finally, the MHM is more versatile for the adsorption of ions in aqueous solution than Fe-Ni/Zn due to its pronounced amphoteric characteristic, which was obtained by βCD incorporation in the inorganic matrix.
Experimental
Materials and Methods
Reagents and ferrite synthesis
βCD was obtained from Xiamen Mchem, Xiamen (China). Salts used for the ferrite synthesis (FeSO4·7H2O, NiSO4·6H2O and ZnSO4·7H2O) were obtained from Merck Laboratory and used without further purification. Magnetic nanoparticles of nickel/zinc (Fe-Ni/Zn) and nickel/zinc/cyclodextrin (Fe-Ni/Zn/βCD) were prepared by coprecipitation reaction of their metal sulfates at 80 °C and pH > 12 (with a sodium hydroxide concentration of 15 g L–1), following the method described in the literature [21]. In the Fe-Ni/Zn/βCD synthesis, 5.0 g L–1 of βCD was used during the preparation process. Solid magnetic materials obtained were washed with hot distilled water and filtered, and then the freeze-dried material was used in the solid-state characterization. Part of the ferrite was kept in water to investigate the properties of the aqueous suspension.
Microscopy analysis
Scanning electron microscopy (SEM) was performed in a JEOL, JSM 840A at 4–10 KV in which samples were covered with a thin gold layer, for electronic contrast. Transmission electron microscopy (TEM) images were obtained in a FEI TECNAI G2 with a thermo-ionic gun at 200 kV. Atomic force microscopy (AFM) images were obtained with a Nanoscope IV MultiMode from Veeco Instruments operating in intermittent contact (tapping) mode, with standard Si probes. Phase-contrast images were acquired simultaneously with topographic images by monitoring, with a lock-in amplifier, the phase lag between the oscillation driver and the actual response of the cantilever.
Dynamic light scattering
Fe-Ni/Zn and Fe-Ni/Zn/βCD average hydrodynamic diameter was measured in a Malvern Zetasizer Nano Series ZS particle analyzer, by using polyethylene square cells. All suspensions were prepared by using Milli-Q water before and after the sonication process by using a Sonics Vibra Cell coupled with a microprobe, at 25% amplitude for 5 min. Samples were measured by monochromatic light (10 mW He–Ne laser, wavelength 632.4 nm) and the scattered light intensity was measured in an angle of 173°. Hydrodynamic diameters were measured five times independently and each one was obtained as the mean of 30 counts.
Gas adsorption/desorption isotherm studies
Textural characteristics of Fe-Ni/Zn or Fe-Ni/Zn/βCD samples were determined through nitrogen gas adsorption (Autosorb–Quantachrome Nova 1200) at liquid-nitrogen temperature. Nitrogen gas was used with a 25-point adsorption–desorption cycle. Samples were outgassed at 100 °C for 3 h before each analysis, and experiments were carried out in triplicate. The specific surface area and fractal dimension were obtained by the application of the Brunauer–Emmett–Teller (BET) equation and the Neimark–Kiselev (NK) methods.
Positron-annihilation-lifetime spectroscopy
PALS measurements of Fe-Ni/Zn or Fe-Ni/Zn/βCD were performed at 294 K by using a conventional fast–fast coincidence system (Ortec) with resolution time of 260 ps given by the 60Co prompt curve. The 22Na positron source, with approximately 20.0 µCi activity, was sandwiched between two 7.6 μm thick kapton foils, and the source correction was approximately 10%. The lifetime spectra (minimum of three measurements per sample) were satisfactorily resolved into three components by the Positronfit-Extended program [45], leading to intensities Ii and lifetimes τi. Subscript i = 1, 2, and 3 refers to p-Ps, e+, and o-Ps, respectively.
Potentiometric titrations
Potentiometric titrations were performed in duplicate with a potentiometric system coupled with glass electrode at 25.0 °C. Each titration experiment consisted of approximately 130 successive injections of a concentrated aqueous solution of HNO3 (14.0 mol L–1) in a beaker loaded with 200 mL of a Fe-Ni/Zn or Fe-Ni/Zn/βCD basic aqueous suspension at an approximate pH of 13.0. The equivalence point was calculated by first-derivative method, which is well established in the literature [46].
Zeta potential
ZP measurements were carried out in a Malvern Zetasizer Nano Series ZS (Malvern Instruments, UK) with a 633 nm red laser, through the Malvern Standard M3 technique (with Doppler electrophoresis as the basic principle of operation) by using capillary cell (DPS1060) [37,47]. Average of the ZP values was calculated by ten independent measurements, each one obtained as the mean of 30 counts. ZP values were measured as a function of pH to evaluate the colloidal stability and these measurements were recorded by using different concentrations of nitric acid or sodium hydroxide. ZP titrations were also used to investigate the adsorption process, in which 51 injections of 5.0 μL increments of Cr(NO3)3 or K2Cr2O7 at 50.0 mmol L–1 aqueous solution were titrated into 10.0 mL of Fe-Ni/Zn or Fe-Ni/Zn/βCD at concentrations of 5.1 mg L–1 and 4.6 mg L–1, respectively. The pH of each solution was not regulated, since the net interaction between the ferrites and the heavy metal could have been disturbed, and moreover, using raw solutions would better represent the conditions in a practical application [4].
Sedimentation studies
The kinetic stability of the suspensions was evaluated by relative turbidity determinations as a function of time by using a FEMTO UV–visible spectrophotometer, in the wavelength of 700 nm and with a 1 cm light path quartz cell. Optical obscuration was recorded in intervals of 4 min over 120 min. Suspensions containing 4.6 mg L–1 of solid ferrite and 5.1 mg L–1 of solid MHM were analyzed at different pH values.
Supporting Information
Supporting Information File 1: Solid-state characterization of the magnetic hybrid materials. | ||
Format: PDF | Size: 441.0 KB | Download |
References
-
Bar-Nahum, I.; Narasimhulu, K. V.; Weiner, L.; Neumann, R. Inorg. Chem. 2005, 44, 4900–4902. doi:10.1021/ic050473c
Return to citation in text: [1] -
Hayashi, K.; Sakamoto, W.; Yogo, T. J. Magn. Magn. Mater. 2009, 321, 450–457. doi:10.1016/j.jmmm.2008.10.004
Return to citation in text: [1] [2] -
Dolbecq, A.; Dumas, E.; Mayer, C. R.; Mialane, P. Chem. Rev. 2010, 110, 6009–6048. doi:10.1021/cr1000578
Return to citation in text: [1] -
Wang, Y.; Cheng, R.; Wen, Z.; Zhao, L. Eur. J. Inorg. Chem. 2011, 2011, 2942–2947. doi:10.1002/ejic.201100205
Return to citation in text: [1] [2] [3] [4] [5] -
Hong, R. Y.; Feng, B.; Chen, L. L.; Liu, G. H.; Li, H. Z.; Zeng, Y.; Wei, D. G. Bio. Chem. Eng. J. 2008, 42, 290–300. doi:10.1016/j.bej.2008.07.009
Return to citation in text: [1] -
Zeng, H.; Sun, S. Adv. Funct. Mater. 2008, 18, 391–400. doi:10.1002/adfm.200701211
Return to citation in text: [1] -
Bocanegra-Diaz, A.; Mohallem, N. D. S.; Novak, M. A.; Sinisterra, R. D. J. Magn. Magn. Mater. 2004, 272–276, 2395–2397. doi:10.1016/j.jmmm.2003.12.975
Return to citation in text: [1] [2] -
Wu, R.; Qu, J.; Chen, Y. Water Res. 2005, 39, 630–638. doi:10.1016/j.watres.2004.11.005
Return to citation in text: [1] -
Chung, H. J.; Lee, H.; Bae, K. H.; Lee, Y.; Park, J.; Cho, S.-W.; Hwang, J. Y.; Park, H.; Langer, R.; Anderson, D.; Park, T. G. ACS Nano 2011, 5, 4329–4336. doi:10.1021/nn201198f
Return to citation in text: [1] -
Jang, J.-t.; Nah, H.; Lee, J.-H.; Moon, S. H.; Kim, M. G.; Cheon, J. Angew. Chem., Int. Ed. 2009, 48, 1234–1238. doi:10.1002/anie.200805149
Return to citation in text: [1] -
Cornell, R. M.; Schwertmann, U. The Iron Oxides: Structure, Properties, Reactions, Occurences and Uses, 2nd ed.; Wiley-VCH: Weinheim, 2003.
Return to citation in text: [1] -
De Sousa, F. B.; Guerreiro, J. D. T.; Ma, M.; Anderson, D. G.; Drum, C. L.; Sinisterra, R. D.; Langer, R. J. Mater. Chem. 2010, 20, 9910–9917. doi:10.1039/c0jm01903h
Return to citation in text: [1] -
Harada, A.; Kobayashi, R.; Takashima, Y.; Hashidzume, A.; Yamaguchi, H. Nat. Chem. 2011, 3, 34–37. doi:10.1038/NCHEM.893
Return to citation in text: [1] -
Lula, I.; De Sousa, F. B.; Denadai, Â. M. L.; Ianzer, D.; Camargo, A. C. M.; Santos, R. A. S.; Sinisterra, R. D. J. Braz. Chem. Soc. 2011, 22, 1765–1773. doi:10.1590/S0103-50532011000900020
Return to citation in text: [1] -
Dos Santos, H. F.; Duarte, H. A.; Sinisterra, R. D.; De Melo Mattos, S. V.; De Oliveira, L. F. C.; De Almeida, W. B. Chem. Phys. Lett. 2000, 319, 569–575. doi:10.1016/S0009-2614(00)00087-7
Return to citation in text: [1] -
Hedges, A. R. Chem. Rev. 1998, 98, 2035–2044. doi:10.1021/cr970014w
Return to citation in text: [1] -
Loftsson, T.; Brewster, M. E. J. Pharm. Sci. 1996, 85, 1017–1025. doi:10.1021/js950534b
Return to citation in text: [1] -
De Sousa, F. B.; Lima, A. C.; Denadai, Â. M. L.; Anconi, C. P. A.; De Almeida, W. B.; Novato, W. T. G.; Dos Santos, H. F.; Drum, C. L.; Langer, R.; Sinisterra, R. D. Phys. Chem. Chem. Phys. 2012, 14, 1934–1944. doi:10.1039/c2cp22768a
Return to citation in text: [1] -
Loftsson, T.; Masson, M.; Brewster, M. E. J. Pharm. Sci. 2004, 93, 1091–1099. doi:10.1002/jps.20047
Return to citation in text: [1] [2] -
Messner, M.; Kurkov, S. V.; Flavia-Piera, R.; Brewster, M. E.; Loftsson, T. Int. J. Pharm. 2011, 408, 235–247. doi:10.1016/j.ijpharm.2011.02.008
Return to citation in text: [1] [2] -
Bocanegra-Diaz, A.; Mohallem, N. D. S.; Sinisterra, R. D. J. Braz. Chem. Soc. 2003, 14, 936–941. doi:10.1590/S0103-50532003000600011
Return to citation in text: [1] [2] -
Kim, D.-H.; Kim, K.-N.; Kim, K. M.; Lee, Y.-K. J. Biomed. Mater. Res., Part A 2009, 88, 1–11. doi:10.1002/jbm.a.31775
Return to citation in text: [1] -
Pongpeerapat, A.; Wanawongthai, C.; Tozuka, Y.; Moribe, K.; Yamamoto, K. Int. J. Pharm. 2008, 352, 309–316. doi:10.1016/j.ijpharm.2007.10.052
Return to citation in text: [1] -
Quickel, T. E.; Le, V. H.; Brezesinski, T.; Tolbert, S. H. Nano Lett. 2010, 10, 2982–2988. doi:10.1021/nl1014266
Return to citation in text: [1] -
Peddis, D.; Cannas, C.; Musinu, A.; Piccaluga, G. Chemistry 2009, 15, 7822–7829. doi:10.1002/chem.200802513
Return to citation in text: [1] -
Miretzky, P.; Fernandez Cirelli, A. J. Hazard. Mater. 2010, 180, 1–19. doi:10.1016/j.jhazmat.2010.04.060
Return to citation in text: [1] -
Denadai, Â. M. L.; Santoro, M. M.; Texeira, A. V.; Sinisterra, R. D. Mater. Sci. Eng., C 2010, 30, 417–422. doi:10.1016/j.msec.2009.12.008
Return to citation in text: [1] -
De Sousa, F. B.; Denadai, Â. M. L.; Lula, I. S.; Nascimento, C. S., Jr.; Fernandes Neto, N. S. G.; Lima, A. C.; De Almeida, W. B.; Sinisterra, R. D. J. Am. Chem. Soc. 2008, 130, 8426–8436. doi:10.1021/ja801080v
Return to citation in text: [1] -
Bonini, M.; Ross, S.; Karlsson, G.; Almgren, M.; Nostro, P. L.; Baglioni, P. Langmuir 2006, 22, 1478–1484. doi:10.1021/la052878f
Return to citation in text: [1] -
Boström, M.; Deniz, V.; Franks, G. V.; Ninham, B. W. Adv. Colloid Interface Sci. 2006, 123–126, 5–15. doi:10.1016/j.cis.2006.05.001
Return to citation in text: [1] -
He, Y.; Ye, T.; Su, M.; Zhang, C.; Ribbe, A. E.; Jiang, W.; Mao, C. Nature 2008, 452, 198–U41. doi:10.1038/nature06597
Return to citation in text: [1] -
Ooya, T.; Huh, K. M.; Saitoh, M.; Tamiya, E.; Park, K. Sci. Technol. Adv. Mater. 2005, 6, 452–456. doi:10.1016/j.stam.2005.01.006
Return to citation in text: [1] -
Banaresmunoz, M. A.; Escribano, V. S. Langmuir 1991, 7, 1779–1783. doi:10.1021/la00056a034
Return to citation in text: [1] -
Tao, S. J. J. Chem. Phys. 1972, 56, 5499. doi:10.1063/1.1677067
Return to citation in text: [1] -
Jean, Y. C. Macromolecules 1996, 29, 5756–5757. doi:10.1021/ma960085h
Return to citation in text: [1] -
Levine, I. N. Physical Chemistry, 4th ed.; McGraw-Hill: New York, 1995.
Return to citation in text: [1] -
Xu, R. Langmuir 1993, 9, 2955–2962. doi:10.1021/la00035a037
Return to citation in text: [1] [2] -
Beattie, J. K. Lab Chip 2006, 6, 1409–1411. doi:10.1039/b610537h
Return to citation in text: [1] -
Collins, K. D. Methods 2004, 34, 300–311. doi:10.1016/j.ymeth.2004.03.021
Return to citation in text: [1] -
Plaza, R. C.; de Vicente, J.; Gomez-Lopera, S.; Delgado, A. V. J. Colloid Interface Sci. 2001, 242, 306–313. doi:10.1006/jcis.2001.7882
Return to citation in text: [1] [2] [3] -
Elimelech, M.; Chen, W. H.; Waypa, J. J. Desalination 1994, 95, 269–286. doi:10.1016/0011-9164(94)00064-6
Return to citation in text: [1] -
Fonseca, C. G.; Basaglia, R. M. F.; Brant, M. C.; Matencio, T.; Domingues, R. Z. Powder Technol. 2009, 192, 352–358. doi:10.1016/j.powtec.2009.01.022
Return to citation in text: [1] -
Rao, K. H.; Forssberg, K. S. E.; Forsling, W. Colloids Surf., A 1998, 133, 107–117. doi:10.1016/S0927-7757(97)00130-1
Return to citation in text: [1] -
Barale, M.; Lefevre, G.; Carrette, F.; Catalette, H.; Fédoroff, M.; Cote, G. J. Colloid Interface Sci. 2008, 328, 34–40. doi:10.1016/j.jcis.2008.09.007
Return to citation in text: [1] -
Kirkegaard, P.; Eldrup, M.; Mogensen, O. E.; Pedersen, N. J. Comput. Phys. Commun. 1981, 23, 307–335. doi:10.1016/0010-4655(81)90006-0
Return to citation in text: [1] -
Mendham, J.; Denney, R. C.; Barnes, J. D.; Thomas, M. J. K. Vogel's Quantitative Chemical Analysis, 6th ed.; Pearson Education Limited: Essex, 2000.
Return to citation in text: [1] -
Tantra, R.; Schulze, P.; Quincey, P. Particuology 2010, 8, 279–285. doi:10.1016/j.partic.2010.01.003
Return to citation in text: [1]
40. | Plaza, R. C.; de Vicente, J.; Gomez-Lopera, S.; Delgado, A. V. J. Colloid Interface Sci. 2001, 242, 306–313. doi:10.1006/jcis.2001.7882 |
41. | Elimelech, M.; Chen, W. H.; Waypa, J. J. Desalination 1994, 95, 269–286. doi:10.1016/0011-9164(94)00064-6 |
42. | Fonseca, C. G.; Basaglia, R. M. F.; Brant, M. C.; Matencio, T.; Domingues, R. Z. Powder Technol. 2009, 192, 352–358. doi:10.1016/j.powtec.2009.01.022 |
43. | Rao, K. H.; Forssberg, K. S. E.; Forsling, W. Colloids Surf., A 1998, 133, 107–117. doi:10.1016/S0927-7757(97)00130-1 |
40. | Plaza, R. C.; de Vicente, J.; Gomez-Lopera, S.; Delgado, A. V. J. Colloid Interface Sci. 2001, 242, 306–313. doi:10.1006/jcis.2001.7882 |
1. | Bar-Nahum, I.; Narasimhulu, K. V.; Weiner, L.; Neumann, R. Inorg. Chem. 2005, 44, 4900–4902. doi:10.1021/ic050473c |
7. | Bocanegra-Diaz, A.; Mohallem, N. D. S.; Novak, M. A.; Sinisterra, R. D. J. Magn. Magn. Mater. 2004, 272–276, 2395–2397. doi:10.1016/j.jmmm.2003.12.975 |
22. | Kim, D.-H.; Kim, K.-N.; Kim, K. M.; Lee, Y.-K. J. Biomed. Mater. Res., Part A 2009, 88, 1–11. doi:10.1002/jbm.a.31775 |
23. | Pongpeerapat, A.; Wanawongthai, C.; Tozuka, Y.; Moribe, K.; Yamamoto, K. Int. J. Pharm. 2008, 352, 309–316. doi:10.1016/j.ijpharm.2007.10.052 |
24. | Quickel, T. E.; Le, V. H.; Brezesinski, T.; Tolbert, S. H. Nano Lett. 2010, 10, 2982–2988. doi:10.1021/nl1014266 |
25. | Peddis, D.; Cannas, C.; Musinu, A.; Piccaluga, G. Chemistry 2009, 15, 7822–7829. doi:10.1002/chem.200802513 |
37. | Xu, R. Langmuir 1993, 9, 2955–2962. doi:10.1021/la00035a037 |
47. | Tantra, R.; Schulze, P.; Quincey, P. Particuology 2010, 8, 279–285. doi:10.1016/j.partic.2010.01.003 |
2. | Hayashi, K.; Sakamoto, W.; Yogo, T. J. Magn. Magn. Mater. 2009, 321, 450–457. doi:10.1016/j.jmmm.2008.10.004 |
5. | Hong, R. Y.; Feng, B.; Chen, L. L.; Liu, G. H.; Li, H. Z.; Zeng, Y.; Wei, D. G. Bio. Chem. Eng. J. 2008, 42, 290–300. doi:10.1016/j.bej.2008.07.009 |
6. | Zeng, H.; Sun, S. Adv. Funct. Mater. 2008, 18, 391–400. doi:10.1002/adfm.200701211 |
4. | Wang, Y.; Cheng, R.; Wen, Z.; Zhao, L. Eur. J. Inorg. Chem. 2011, 2011, 2942–2947. doi:10.1002/ejic.201100205 |
26. | Miretzky, P.; Fernandez Cirelli, A. J. Hazard. Mater. 2010, 180, 1–19. doi:10.1016/j.jhazmat.2010.04.060 |
4. | Wang, Y.; Cheng, R.; Wen, Z.; Zhao, L. Eur. J. Inorg. Chem. 2011, 2011, 2942–2947. doi:10.1002/ejic.201100205 |
4. | Wang, Y.; Cheng, R.; Wen, Z.; Zhao, L. Eur. J. Inorg. Chem. 2011, 2011, 2942–2947. doi:10.1002/ejic.201100205 |
18. | De Sousa, F. B.; Lima, A. C.; Denadai, Â. M. L.; Anconi, C. P. A.; De Almeida, W. B.; Novato, W. T. G.; Dos Santos, H. F.; Drum, C. L.; Langer, R.; Sinisterra, R. D. Phys. Chem. Chem. Phys. 2012, 14, 1934–1944. doi:10.1039/c2cp22768a |
19. | Loftsson, T.; Masson, M.; Brewster, M. E. J. Pharm. Sci. 2004, 93, 1091–1099. doi:10.1002/jps.20047 |
20. | Messner, M.; Kurkov, S. V.; Flavia-Piera, R.; Brewster, M. E.; Loftsson, T. Int. J. Pharm. 2011, 408, 235–247. doi:10.1016/j.ijpharm.2011.02.008 |
45. | Kirkegaard, P.; Eldrup, M.; Mogensen, O. E.; Pedersen, N. J. Comput. Phys. Commun. 1981, 23, 307–335. doi:10.1016/0010-4655(81)90006-0 |
2. | Hayashi, K.; Sakamoto, W.; Yogo, T. J. Magn. Magn. Mater. 2009, 321, 450–457. doi:10.1016/j.jmmm.2008.10.004 |
3. | Dolbecq, A.; Dumas, E.; Mayer, C. R.; Mialane, P. Chem. Rev. 2010, 110, 6009–6048. doi:10.1021/cr1000578 |
21. | Bocanegra-Diaz, A.; Mohallem, N. D. S.; Sinisterra, R. D. J. Braz. Chem. Soc. 2003, 14, 936–941. doi:10.1590/S0103-50532003000600011 |
46. | Mendham, J.; Denney, R. C.; Barnes, J. D.; Thomas, M. J. K. Vogel's Quantitative Chemical Analysis, 6th ed.; Pearson Education Limited: Essex, 2000. |
4. | Wang, Y.; Cheng, R.; Wen, Z.; Zhao, L. Eur. J. Inorg. Chem. 2011, 2011, 2942–2947. doi:10.1002/ejic.201100205 |
12. | De Sousa, F. B.; Guerreiro, J. D. T.; Ma, M.; Anderson, D. G.; Drum, C. L.; Sinisterra, R. D.; Langer, R. J. Mater. Chem. 2010, 20, 9910–9917. doi:10.1039/c0jm01903h |
13. | Harada, A.; Kobayashi, R.; Takashima, Y.; Hashidzume, A.; Yamaguchi, H. Nat. Chem. 2011, 3, 34–37. doi:10.1038/NCHEM.893 |
4. | Wang, Y.; Cheng, R.; Wen, Z.; Zhao, L. Eur. J. Inorg. Chem. 2011, 2011, 2942–2947. doi:10.1002/ejic.201100205 |
10. | Jang, J.-t.; Nah, H.; Lee, J.-H.; Moon, S. H.; Kim, M. G.; Cheon, J. Angew. Chem., Int. Ed. 2009, 48, 1234–1238. doi:10.1002/anie.200805149 |
14. | Lula, I.; De Sousa, F. B.; Denadai, Â. M. L.; Ianzer, D.; Camargo, A. C. M.; Santos, R. A. S.; Sinisterra, R. D. J. Braz. Chem. Soc. 2011, 22, 1765–1773. doi:10.1590/S0103-50532011000900020 |
15. | Dos Santos, H. F.; Duarte, H. A.; Sinisterra, R. D.; De Melo Mattos, S. V.; De Oliveira, L. F. C.; De Almeida, W. B. Chem. Phys. Lett. 2000, 319, 569–575. doi:10.1016/S0009-2614(00)00087-7 |
16. | Hedges, A. R. Chem. Rev. 1998, 98, 2035–2044. doi:10.1021/cr970014w |
17. | Loftsson, T.; Brewster, M. E. J. Pharm. Sci. 1996, 85, 1017–1025. doi:10.1021/js950534b |
21. | Bocanegra-Diaz, A.; Mohallem, N. D. S.; Sinisterra, R. D. J. Braz. Chem. Soc. 2003, 14, 936–941. doi:10.1590/S0103-50532003000600011 |
9. | Chung, H. J.; Lee, H.; Bae, K. H.; Lee, Y.; Park, J.; Cho, S.-W.; Hwang, J. Y.; Park, H.; Langer, R.; Anderson, D.; Park, T. G. ACS Nano 2011, 5, 4329–4336. doi:10.1021/nn201198f |
40. | Plaza, R. C.; de Vicente, J.; Gomez-Lopera, S.; Delgado, A. V. J. Colloid Interface Sci. 2001, 242, 306–313. doi:10.1006/jcis.2001.7882 |
8. | Wu, R.; Qu, J.; Chen, Y. Water Res. 2005, 39, 630–638. doi:10.1016/j.watres.2004.11.005 |
11. | Cornell, R. M.; Schwertmann, U. The Iron Oxides: Structure, Properties, Reactions, Occurences and Uses, 2nd ed.; Wiley-VCH: Weinheim, 2003. |
44. | Barale, M.; Lefevre, G.; Carrette, F.; Catalette, H.; Fédoroff, M.; Cote, G. J. Colloid Interface Sci. 2008, 328, 34–40. doi:10.1016/j.jcis.2008.09.007 |
29. | Bonini, M.; Ross, S.; Karlsson, G.; Almgren, M.; Nostro, P. L.; Baglioni, P. Langmuir 2006, 22, 1478–1484. doi:10.1021/la052878f |
7. | Bocanegra-Diaz, A.; Mohallem, N. D. S.; Novak, M. A.; Sinisterra, R. D. J. Magn. Magn. Mater. 2004, 272–276, 2395–2397. doi:10.1016/j.jmmm.2003.12.975 |
19. | Loftsson, T.; Masson, M.; Brewster, M. E. J. Pharm. Sci. 2004, 93, 1091–1099. doi:10.1002/jps.20047 |
20. | Messner, M.; Kurkov, S. V.; Flavia-Piera, R.; Brewster, M. E.; Loftsson, T. Int. J. Pharm. 2011, 408, 235–247. doi:10.1016/j.ijpharm.2011.02.008 |
27. | Denadai, Â. M. L.; Santoro, M. M.; Texeira, A. V.; Sinisterra, R. D. Mater. Sci. Eng., C 2010, 30, 417–422. doi:10.1016/j.msec.2009.12.008 |
28. | De Sousa, F. B.; Denadai, Â. M. L.; Lula, I. S.; Nascimento, C. S., Jr.; Fernandes Neto, N. S. G.; Lima, A. C.; De Almeida, W. B.; Sinisterra, R. D. J. Am. Chem. Soc. 2008, 130, 8426–8436. doi:10.1021/ja801080v |
33. | Banaresmunoz, M. A.; Escribano, V. S. Langmuir 1991, 7, 1779–1783. doi:10.1021/la00056a034 |
34. | Tao, S. J. J. Chem. Phys. 1972, 56, 5499. doi:10.1063/1.1677067 |
35. | Jean, Y. C. Macromolecules 1996, 29, 5756–5757. doi:10.1021/ma960085h |
30. | Boström, M.; Deniz, V.; Franks, G. V.; Ninham, B. W. Adv. Colloid Interface Sci. 2006, 123–126, 5–15. doi:10.1016/j.cis.2006.05.001 |
31. | He, Y.; Ye, T.; Su, M.; Zhang, C.; Ribbe, A. E.; Jiang, W.; Mao, C. Nature 2008, 452, 198–U41. doi:10.1038/nature06597 |
32. | Ooya, T.; Huh, K. M.; Saitoh, M.; Tamiya, E.; Park, K. Sci. Technol. Adv. Mater. 2005, 6, 452–456. doi:10.1016/j.stam.2005.01.006 |
© 2012 Denadai et al; licensee Beilstein-Institut.
This is an Open Access article under the terms of the Creative Commons Attribution License (http://creativecommons.org/licenses/by/2.0), which permits unrestricted use, distribution, and reproduction in any medium, provided the original work is properly cited.
The license is subject to the Beilstein Journal of Organic Chemistry terms and conditions: (http://www.beilstein-journals.org/bjoc)