Abstract
Friedel–Crafts-type reaction of pyrene with diethyl 1-(isothiocyanato)alkylphosphonates promoted by trifluoromethanosulfonic acid afforded diethyl 1-(pyrene-1-carbothioamido)alkylphosphonates in 83–94% yield. These compounds were transformed, in 87–94% yield, into the corresponding diethyl 1-(pyrene-1-carboxamido)alkylphosphonates by treatment with Oxone®. 1-(Pyrene-1-carboxamido)methylphosphonic acid was obtained in a 87% yield by treating the corresponding diethyl phosphonate with Me3Si-Br in methanol. All of the synthesized amidophosphonates were emissive in solution and in the solid state. The presence of a phosphonato group brought about an approximately two-fold increase in solution fluorescence quantum yield in comparison with that of a model N-alkyl pyrene-1-carboxamide. This effect was tentatively explained by stiffening of the amidophosphonate lateral chain which was caused by the interaction (intramolecular hydrogen bond) of phosphonate and amide groups. The synthesized phosphonic acid was soluble in a biological aqueous buffer (PBS, 0.01 M, pH 7.35) and was strongly emissive under these conditions (λem = 383, 400 nm, τ = 18.7 ns, ΦF > 0.98). Solid-state emission of diethyl 1-(pyrene-1-carboxamido)methylphosphonate (λmax = 485 nm; ΦF = 0.25) was assigned to π–π aggregates, the presence of which was revealed by single-crystal X-ray diffraction analysis.
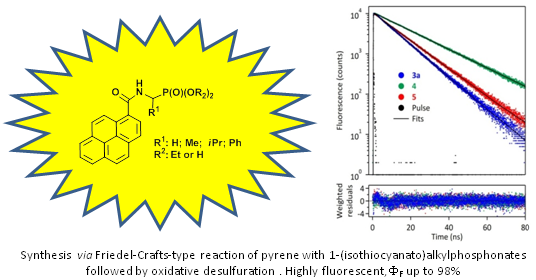
Graphical Abstract
Introduction
Friedel–Crafts-type reaction of arenes with isothiocyanates constitutes a useful method for the synthesis of aromatic secondary thioamides [1-6]. Our group [7] and others [8] have recently described an efficient modification of this method by using trifluoromethanesulfonic (triflic) acid as a promoter. Furthermore, we reported a simple procedure for the oxidative desulfurization of thioamides to amides via reaction with Oxone®. Now we want to apply this approach to the synthesis of N-thioacyl- and acyl derivatives of 1-aminoalkylphosphonates from arenes and 1-(isothiocyanato)alkylphosphonates. 1-Aminoalkylphosphonates and their derivatives are compounds of biological relevance which have attracted the interest of biologically-oriented chemists and biochemists [9-12]. Furthermore, the phosphonate group offers various possibilities of metal binding or anchoring to solid surfaces [13-20]. For our purposes we chose pyrene as a model arene because of its high reactivity in reaction with simple isothiocyanates [7], and because the expected products, 1-(pyrene-1-carbothioamido)alkylphosphonates, should be easily transformed into the corresponding fluorescent amides [7,21-28]. Herein we report the results of this study along with the photophysical properties of the synthesized 1-(pyrene-1-carboxamido)alkylphosphonates.
Results and Discussion
Syntheses
The reactions performed in this work are shown in Scheme 1.
Scheme 1: Synthesis of diethyl 1-(pyrene-1-carbothioamido)alkylphosphonates 2a–d, diethyl 1-(pyrene-1-carboxamido)alkylphosphonates 3a–d and (pyrene-1-carboxamido)methylphosphonic acid (4).
Scheme 1: Synthesis of diethyl 1-(pyrene-1-carbothioamido)alkylphosphonates 2a–d, diethyl 1-(pyrene-1-carboxa...
We found that pyrene reacts with isothiocyanates 1a–d in the presence of trifluoromethanesulfonic acid (TfOH) in dichloromethane at room temperature to afford 1-(pyrene-1-carbothioamido)alkylphosphonates 2a–d in high (83–94%) isolated yields. These yields are comparable with those obtained using simple alkyl isothiocyanates [7], which means that the phosphonato group is perfectly compatible with the reaction conditions. The structures of 2a–d were confirmed by spectroscopic and elemental analysis data and (for 3a) by a single-crystal X-ray diffraction study (vide infra).
Thioamidophoshonates 2a–d readily reacted with Oxone® in acetonitrile–water at room temperature to afford the corresponding amidophosphonates 3a–d in 87–94% isolated yield (Scheme 1). Therefore, the indirect route to these compounds described above proved very efficient. We did not attempt to prepare 3a–d via direct Friedel–Crafts-type reaction of pyrene with (isocyanato)alkyl phosphonates because these compounds are difficult to synthesize and unstable [29]. Moreover, in our recent work we observed that reaction of pyrene with isothiocyanates proceeds more efficiently than an analogous reaction with isocyanates [7].
Additionally, compound 3a was transformed, using a standard procedure [30], to the corresponding phosphonic acid 4 isolated in 87% yield.
Photophysical properties of 3a–d and 4
As expected, thioamides 2a–d were nonfluorescent (thioamide group is a well-known fluorescence quencher [31]). In contrast, the corresponding amides 3a–d showed strong fluorescence emission in solution and in the solid state. We studied the photophysical properties of 3a–d and 4 and, for comparison, those of N-alkylamide 5 (Figure 1).
The steady-state spectroscopic data for 3a–d, 4 and 5, along with fluorescence quantum yields in chloroform solutions, are presented in Table 1 (although it was recently reported that pyrene undergoes photodecomposition in this solvent [32], we found that this did not happen in the case of the compounds investigated here).
Table 1: Steady-state absorption and emission data for 3a–d, 4, 5 in CHCl3 solutions (c = 10−6 M).
Compound | Absorption λmax/nm (εmax/M−1 cm−1) | Emissionb λmax/nm | ΦF |
---|---|---|---|
3a | 331 (27450), 345 (50910), 380 (960) | 385, 405 | 0.68 |
3b | 332 (38710), 345 (52480), 379 (4000) | 385, 404 | 0.66 |
3c | 331 (37850), 345 (51610), 379 (2750) | 385, 404 | 0.63 |
3d | 331 (37220), 345 (51400), 379 (4200) | 387, 406 | 0.68 |
4a | 327 (36350), 341 (50410), 376 (5100) | 383, 400 | >0.98 |
5 | 316 (16990), 329 (37400), 345 (52360), 377 (2030) | 385, 403 | 0.34 |
a10–6 M solution in 0.01 M PBS (pH 7.35). bλexcit = 360 nm.
Electronic absorption and emission spectra of 3a in various solvents are shown in Figure 2.
![[1860-5397-11-266-2]](/bjoc/content/figures/1860-5397-11-266-2.png?scale=2.0&max-width=1024&background=FFFFFF)
Figure 2: Normalized electronic absorption (a) and emission (b) spectra of 3a in various solvents.
Figure 2: Normalized electronic absorption (a) and emission (b) spectra of 3a in various solvents.
The introduction of a phosphonato group into the N-alkyl chain of the pyrene carboxamide fluorophore practically did not influence the electronic absorption and emission spectra. Structured emission bands were observed showing only a small effect of solvent polarity (Figure 1). However, the fluorescence quantum yields of 3a–d and 4 were significantly higher than that of 5.
In order to explain this phenomenon we performed a time-resolved fluorescence study of 3a, 4 and 5. The fluorescence decay curves are shown in Figure 3, whereas the fluorescence lifetimes and decay rate constants are presented in Table 2. All of the compounds under study displayed monoexponential decays, thus indicating one emitting species.
![[1860-5397-11-266-3]](/bjoc/content/figures/1860-5397-11-266-3.png?scale=2.0&max-width=1024&background=FFFFFF)
Figure 3: Fluorescence decay curves for 3a and 5 in chloroform and for 4 in 0.01 M PBS (pH 7.35). λexcit = 360 nm; λem = 385, 390 and 383 nm, respectively.
Figure 3: Fluorescence decay curves for 3a and 5 in chloroform and for 4 in 0.01 M PBS (pH 7.35). λexcit = 36...
Table 2: Fluorescence lifetimes and decay rate constants for 3a and 5 in CHCl3 and for 4 in 0.01 M PBS (pH 7.35).
Compound | τ (ns); contribution | kra 107 s−1 | knrb 107 s−1 |
---|---|---|---|
3a | 10.6 (1.00) | 6.4 | 3.0 |
4 | 18.7 (1.00) | 5.2 | 0.1 |
5 | 12.3 (1.00) | 2.8 | 5.4 |
akr = ΦF /τ; bknr = (1−ΦF )/τ.
Table 2 shows that 3a displays a slightly shorter excited state lifetime (10.6 ns) than its alkyl analogue 5 (12.3 ns). The higher emission quantum yield of 3a results from a ≈2.3 times higher value of kr and a concomitant ca. 1.8 times lower value of knr. Notably, compound 4 displays a kr value that is comparable to that of 3a (kr = 5.3–6.4 × 107 s−1 for the two species), but its non-radiative deactivation pathway is almost entirely suppressed. Therefore, it may be concluded that the phosphonato group exerts a significant influence on the excited state deactivation kinetics of 3a.
This influence may be attributed to the highly polar nature of the phosphonato group (electrostatic interaction between C=O and P=O dipoles may hamper rotations in the lateral chain responsible for non-radiative deactivation of the excited state). Another factor to be taken into consideration is the possibility of an intramolecular hydrogen bond between the N–H and P=O groups (Figure 4), which also leads to stiffening of the lateral chain and to blocking the non-radiative deactivation channel.
Figure 4: Intramolecular hydrogen bond in 3a–d.
Figure 4: Intramolecular hydrogen bond in 3a–d.
The presence of such a bond was verified via variable concentration 1H NMR spectroscopy [33], which showed only small variation (≈0.2 ppm) in the chemical shift of the N–H resonance of 3a across a concentration range of 0.1–0.005 M in CDCl3 (see Supporting Information File 1).
Compound 4 was found to be insoluble in chloroform and in other weakly polar and nonpolar organic solvents but soluble in a biological buffer (0.01 M PBS, pH 7.35). Obviously, under these conditions it exists under an ionized form (anion or dianion). As was mentioned earlier, we observed efficient (ΦF > 0.98) and relatively long-lived emission from 4 in the PBS buffer, which promises the possibility of its application in biological research.
Compound 3a was also emissive in the solid state (λmax = 485 nm; ΦF = 0.25). The significant bathochromic shift (80 nm) and the broadness of the emission band in comparison with solution fluorescence (Figure 5) suggest the presence of emissive aggregates in the solid state. This was confirmed by an X-ray diffraction study (vide infra).
![[1860-5397-11-266-5]](/bjoc/content/figures/1860-5397-11-266-5.png?scale=2.0&max-width=1024&background=FFFFFF)
Figure 5: Normalized electronic absorption (violet) and emission (pink) spectrum of 3a in CHCl3 (c = 10−6 M) and its solid-state emission spectrum (yellow). λexcit = 360 nm.
Figure 5: Normalized electronic absorption (violet) and emission (pink) spectrum of 3a in CHCl3 (c = 10−6 M) ...
Molecular structure, crystal packing and solid-state fluorescence of 3a
Compound 3a crystallized from layered dichloromethane–hexane in the monoclinic space group P21/c, with a single molecule occupying an asymmetric unit in general position. Its molecular structure is shown in Figure 6.
![[1860-5397-11-266-6]](/bjoc/content/figures/1860-5397-11-266-6.png?scale=2.0&max-width=1024&background=FFFFFF)
Figure 6: Molecular structure of 3a (ORTEP representation). Displacement ellipsoids were drawn at a 50% probability level. Hydrogen atom labels are analogous to those of bonded non-H atoms and were omitted for clarity, apart from the H1 atom which was involved in intermolecular hydrogen bond formation.
Figure 6: Molecular structure of 3a (ORTEP representation). Displacement ellipsoids were drawn at a 50% proba...
The pyrenyl moiety in 3a is slightly bent, the angle between the planes of rings C1–C2–C15–C14–C13–C12 and C5–C6–C7–C8–C9–C16 is 4.23(13)°. The amide group is twisted by 57.1(2) degrees from the plane of the C1–C2–C15–C14–C13–C12 ring. Together with the C1–C17 bond length exceeding 1.5 Å, this suggests that there is only very weak electronic conjugation between these units. The phosphonato group is also almost perpendicular to the plane of the amide group; the relevant C17–N1–C18–P1 torsion angle is 102.39(13)°. The most important geometrical parameters and intermolecular contacts for the structure are summarized in Table 3 and Table 4, respectively.
Table 3: Selected geometrical parameters for 3a.
Bond lengths | Å |
---|---|
P1–O2 | 1.5761(11) |
P1–O3 | 1.4751(10) |
P1–O4 | 1.5694(11) |
P1–C18 | 1.8000(14) |
C1–C17 | 1.5053(18) |
O1–C17 | 1.2315(16) |
N1–C18 | 1.4500(17) |
N1–C17 | 1.3463(17) |
Torsion angles | ° |
C12–C15–C16–C5 | −179.43(12) |
C12–C15–C16–C9 | −0.42(19) |
C2–C1–C17–O1 | −123.81(15) |
C2–C1–C17–N1 | 58.89(17) |
C17–N1–C18–P1 | 102.39(13) |
Table 4: Selected hydrogen bonds in the crystal structure of 3a.
D | H | A | D–H [Å] | D–H [Å] | D–H [Å] | D–H … A [ ° ] | Symmetry operation for A atom |
---|---|---|---|---|---|---|---|
N1 | H1 | O3 | 0.871(14) | 1.941(14) | 2.7991(15) | 168.0(16) | 1−x, −y, 1−z |
C20 | H20C | O1 | 0.981 | 2.683 | 3.369(2) | 127.3 | 1−x, −½+y, ½−z |
C21 | H21A | O1 | 0.990 | 2.6145 | 3.585(2) | 166.6 | 1−x, −½+y, ½−z |
C22 | H22C | O3 | 0.980 | 2.708 | 3.664(2) | 165.2 | 1−x, ½+y, ½−z |
The molecules 3a form dimers in the crystal related by the crystallographic center of symmetry, bound by intermolecular N1–H1···O3 hydrogen bonds and stabilized by C19–H19B···π interactions. Similar NH···O=P hydrogen-bonded dimers were already observed in the crystals of some aminophosphonates [34].
The dimers form strings along the crystallographic [001] direction, bound by relatively short (H···O distances of less than 2.75 Å) and intermolecular hydrogen C–H···O bonds (Table 4). On the other hand, the pyrenyl moieties from the adjacent strings along [100] take part in π···π interactions, also forming molecular dimers (Figure 7). The shortest C···C distances are C7–C15 and C5–C9 (3.367(2) Å, and 3.410(2) Å, respectively).
![[1860-5397-11-266-7]](/bjoc/content/figures/1860-5397-11-266-7.png?scale=2.0&max-width=1024&background=FFFFFF)
Figure 7: (a) Dimers of π-stacked and hydrogen-bonded molecules of 3a represented in single figures; (b) network of weak C–H…O and C–H…π interactions stabilizing H-bonded dimer strings and (c) crystal packing along the crystallographic b direction. Ellipsoids are drawn at 50% probability level for all non-C and non-H atoms. The remaining atoms are represented as wires in grey or green in order to differentiate between adjacent π…π interacting chains of H-bonded atoms. The shortest intermolecular contacts are represented in cyan.
Figure 7: (a) Dimers of π-stacked and hydrogen-bonded molecules of 3a represented in single figures; (b) netw...
The presence of π–π-bonded aggregates in the crystal structure of 3a is in line with the observed features of solid-state emission of this compound (vide suppra).
Conclusion
We demonstrated the feasibility of the Friedel–Crafts reaction with 1-(isothiocyanato)alkylphosphonates by using pyrene as an arene. The pyrenyl thioamidoalkylphosphonates formed in this reaction can be readily transformed into the corresponding fluorescent amidoalkylphosphonates. It is worthy to note that this class of compounds offers numerous possibilities of chemical transformations [35,36]. The pyrenyl amidoalkylphosphonates emit fluorescence with quantum yields ca. 2 times higher than simple N-substituted pyrene-1-carboxamides. Solid-state emission assigned to the aggregates was also observed. Finally, a pyrenecarboxamide phosphonic acid was synthesized, showing very efficient emission in a biological buffer and promising possible biological applications.
References
-
Arnswald, M.; Neumann, W. P. J. Org. Chem. 1993, 58, 7022–7028. doi:10.1021/jo00077a020
Return to citation in text: [1] -
Jagodziński, T. Org. Prep. Proced. Int. 1990, 22, 755–760. doi:10.1080/00304949009457904
Return to citation in text: [1] -
Papadopoulos, E. P. J. Org. Chem. 1976, 41, 962–965. doi:10.1021/jo00868a013
Return to citation in text: [1] -
Plażuk, D.; Zakrzewski, J.; Rybarczyk-Pirek, A.; Domagala, S. J. Organomet. Chem. 2005, 690, 4302–4308. doi:10.1016/j.jorganchem.2005.06.037
Return to citation in text: [1] -
Tabak, G.; Pham, T.-N.; Levesque, G.; Haraoubia, R. J. Polym. Sci., Part A: Polym. Chem. 1998, 36, 117–127. doi:10.1002/(sici)1099-0518(19980115)36:1<117::aid-pola16>3.0.co;2-m
Return to citation in text: [1] -
Wesołowska, A.; Gros, L.; Westerlich, S.; Jagodziński, T. S. ARKIVOC 2008, No. xv, 239–255.
Return to citation in text: [1] -
Wrona-Piotrowicz, A.; Zakrzewski, J.; Métivier, R.; Brosseau, A.; Makal, A.; Woźniak, K. RSC Adv. 2014, 4, 56003–56012. doi:10.1039/C4RA07045C
Return to citation in text: [1] [2] [3] [4] [5] -
Varun, B. V.; Sood, A.; Prabhu, K. R. RSC Adv. 2014, 4, 60798–60807. doi:10.1039/C4RA12944J
Return to citation in text: [1] -
Berlicki, L.; Kafarski, P. Curr. Org. Chem. 2005, 9, 1829–1850. doi:10.2174/138527205774913088
Return to citation in text: [1] -
Grembecka, J.; Kafarski, P. Mini-Rev. Med. Chem. 2001, 1, 133–144. doi:10.2174/1389557013406990
Return to citation in text: [1] -
Kafarski, P.; Lejczak, B. Phosphorus, Sulfur Silicon Relat. Elem. 1991, 63, 193–215. doi:10.1080/10426509108029443
Return to citation in text: [1] -
Kafarski, P.; Lejczak, B. Curr. Med. Chem. - Anti-Cancer Agents 2001, 1, 301–312. doi:10.2174/1568011013354543
Return to citation in text: [1] -
Goura, J.; Chandrasekhar, V. Chem. Rev. 2015, 115, 6854–6965. doi:10.1021/acs.chemrev.5b00107
Return to citation in text: [1] -
Clearfield, A. Curr. Opin. Solid State Mater. Sci. 2002, 6, 495–506. doi:10.1016/s1359-0286(02)00151-1
Return to citation in text: [1] -
Clearfield, A.; Sharma, C. V. K.; Zhang, B. P. Chem. Mater. 2001, 13, 3099–3112. doi:10.1021/cm010164u
Return to citation in text: [1] -
Gałęzowska, J.; Gumienna-Kontecka, E. Coord. Chem. Rev. 2012, 256, 105–124. doi:10.1016/j.ccr.2011.07.002
Return to citation in text: [1] -
Katz, H. E. Chem. Mater. 1994, 6, 2227–2232. doi:10.1021/cm00048a009
Return to citation in text: [1] -
Mao, J.-G. Coord. Chem. Rev. 2007, 251, 1493–1520. doi:10.1016/j.ccr.2007.02.008
Return to citation in text: [1] -
Shimizu, G. K. H.; Vaidhyanathan, R.; Taylor, J. M. Chem. Soc. Rev. 2009, 38, 1430–1449. doi:10.1039/b802423p
Return to citation in text: [1] -
Tušek-Božić, L. Curr. Med. Chem. 2013, 20, 2096–2117. doi:10.2174/0929867311320160004
Return to citation in text: [1] -
Niko, Y.; Cho, Y.; Kawauchi, S.; Konishi, G.-i. RSC Adv. 2014, 4, 36480–36484. doi:10.1039/C4RA06282E
Return to citation in text: [1] -
Niko, Y.; Konishi, G.-i. Macromolecules 2012, 45, 2327–2337. doi:10.1021/ma3001252
Return to citation in text: [1] -
Niko, Y.; Hiroshige, Y.; Kawauchi, S.; Konishi, G.-i. J. Org. Chem. 2012, 77, 3986–3996. doi:10.1021/jo300317r
Return to citation in text: [1] -
Niko, Y.; Hiroshige, Y.; Kawauchi, S.; Konishi, G.-i. Tetrahedron 2012, 68, 6177–6185. doi:10.1016/j.tet.2012.05.072
Return to citation in text: [1] -
Hwang, J.; Choi, M. G.; Eor, S.; Chang, S.-K. Inorg. Chem. 2012, 51, 1634–1639. doi:10.1021/ic2019428
Return to citation in text: [1] -
Kerr, C. E.; Mitchell, C. D.; Ying, Y.-M.; Eaton, B. E.; Netzel, T. L. J. Phys. Chem. B 2000, 104, 2166–2175. doi:10.1021/jp993776z
Return to citation in text: [1] -
Kerr, C. E.; Mitchell, C. D.; Headrick, J.; Eaton, B. E.; Netzel, T. L. J. Phys. Chem. B 2000, 104, 1637–1650. doi:10.1021/jp992773j
Return to citation in text: [1] -
Frazer, J. D.; Horner, S. M.; Woski, S. A. Tetrahedron Lett. 1998, 39, 1279–1282. doi:10.1016/S0040-4039(97)10853-X
Return to citation in text: [1] -
Coutrot, P.; Grison, C.; Charbonnier-Gérardin, C. Tetrahedron 1992, 48, 9841–9868. doi:10.1016/S0040-4020(01)92278-1
Return to citation in text: [1] -
McKenna, C. E.; Higa, M. T.; Cheung, N. H.; McKenna, M.-C. Tetrahedron Lett. 1977, 18, 155–158. doi:10.1016/S0040-4039(01)92575-4
Return to citation in text: [1] -
Goldberg, J. M.; Wissner, R. F.; Klein, A. M.; Petersson, E. J. Chem. Commun. 2012, 48, 1550–1552. doi:10.1039/c1cc14708k
Return to citation in text: [1] -
Aguilera-Sigalat, J.; Sanchez-SanMartín, J.; Agudelo-Morales, C. E.; Zaballos, E.; Galian, R. E.; Pérez-Prieto, J. ChemPhysChem 2012, 13, 835–844. doi:10.1002/cphc.201100843
Return to citation in text: [1] -
Smith, A. B., III; Ducry, L.; Corbett, R. M.; Hirschmann, R. Org. Lett. 2000, 2, 3887–3890. doi:10.1021/ol0066330
Return to citation in text: [1] -
Juribašić, M.; Bellotto, L.; Tušek-Božić, L. Struct. Chem. 2012, 23, 257–266. doi:10.1007/s11224-011-9859-z
Return to citation in text: [1] -
Katritzky, A. R.; Zhang, G.; Jiang, J. J. Org. Chem. 1994, 59, 4556–4560. doi:10.1021/jo00095a035
Return to citation in text: [1] -
Kuźnik, A.; Mazurkiewicz, R.; Grymel, M.; Zielińska, K.; Adamek, J.; Chmielewska, E.; Bochno, M.; Kubica, S. Beilstein J. Org. Chem. 2015, 11, 1418–1424. doi:10.3762/bjoc.11.153
Return to citation in text: [1]
1. | Arnswald, M.; Neumann, W. P. J. Org. Chem. 1993, 58, 7022–7028. doi:10.1021/jo00077a020 |
2. | Jagodziński, T. Org. Prep. Proced. Int. 1990, 22, 755–760. doi:10.1080/00304949009457904 |
3. | Papadopoulos, E. P. J. Org. Chem. 1976, 41, 962–965. doi:10.1021/jo00868a013 |
4. | Plażuk, D.; Zakrzewski, J.; Rybarczyk-Pirek, A.; Domagala, S. J. Organomet. Chem. 2005, 690, 4302–4308. doi:10.1016/j.jorganchem.2005.06.037 |
5. | Tabak, G.; Pham, T.-N.; Levesque, G.; Haraoubia, R. J. Polym. Sci., Part A: Polym. Chem. 1998, 36, 117–127. doi:10.1002/(sici)1099-0518(19980115)36:1<117::aid-pola16>3.0.co;2-m |
6. | Wesołowska, A.; Gros, L.; Westerlich, S.; Jagodziński, T. S. ARKIVOC 2008, No. xv, 239–255. |
13. | Goura, J.; Chandrasekhar, V. Chem. Rev. 2015, 115, 6854–6965. doi:10.1021/acs.chemrev.5b00107 |
14. | Clearfield, A. Curr. Opin. Solid State Mater. Sci. 2002, 6, 495–506. doi:10.1016/s1359-0286(02)00151-1 |
15. | Clearfield, A.; Sharma, C. V. K.; Zhang, B. P. Chem. Mater. 2001, 13, 3099–3112. doi:10.1021/cm010164u |
16. | Gałęzowska, J.; Gumienna-Kontecka, E. Coord. Chem. Rev. 2012, 256, 105–124. doi:10.1016/j.ccr.2011.07.002 |
17. | Katz, H. E. Chem. Mater. 1994, 6, 2227–2232. doi:10.1021/cm00048a009 |
18. | Mao, J.-G. Coord. Chem. Rev. 2007, 251, 1493–1520. doi:10.1016/j.ccr.2007.02.008 |
19. | Shimizu, G. K. H.; Vaidhyanathan, R.; Taylor, J. M. Chem. Soc. Rev. 2009, 38, 1430–1449. doi:10.1039/b802423p |
20. | Tušek-Božić, L. Curr. Med. Chem. 2013, 20, 2096–2117. doi:10.2174/0929867311320160004 |
34. | Juribašić, M.; Bellotto, L.; Tušek-Božić, L. Struct. Chem. 2012, 23, 257–266. doi:10.1007/s11224-011-9859-z |
9. | Berlicki, L.; Kafarski, P. Curr. Org. Chem. 2005, 9, 1829–1850. doi:10.2174/138527205774913088 |
10. | Grembecka, J.; Kafarski, P. Mini-Rev. Med. Chem. 2001, 1, 133–144. doi:10.2174/1389557013406990 |
11. | Kafarski, P.; Lejczak, B. Phosphorus, Sulfur Silicon Relat. Elem. 1991, 63, 193–215. doi:10.1080/10426509108029443 |
12. | Kafarski, P.; Lejczak, B. Curr. Med. Chem. - Anti-Cancer Agents 2001, 1, 301–312. doi:10.2174/1568011013354543 |
35. | Katritzky, A. R.; Zhang, G.; Jiang, J. J. Org. Chem. 1994, 59, 4556–4560. doi:10.1021/jo00095a035 |
36. | Kuźnik, A.; Mazurkiewicz, R.; Grymel, M.; Zielińska, K.; Adamek, J.; Chmielewska, E.; Bochno, M.; Kubica, S. Beilstein J. Org. Chem. 2015, 11, 1418–1424. doi:10.3762/bjoc.11.153 |
8. | Varun, B. V.; Sood, A.; Prabhu, K. R. RSC Adv. 2014, 4, 60798–60807. doi:10.1039/C4RA12944J |
32. | Aguilera-Sigalat, J.; Sanchez-SanMartín, J.; Agudelo-Morales, C. E.; Zaballos, E.; Galian, R. E.; Pérez-Prieto, J. ChemPhysChem 2012, 13, 835–844. doi:10.1002/cphc.201100843 |
7. | Wrona-Piotrowicz, A.; Zakrzewski, J.; Métivier, R.; Brosseau, A.; Makal, A.; Woźniak, K. RSC Adv. 2014, 4, 56003–56012. doi:10.1039/C4RA07045C |
33. | Smith, A. B., III; Ducry, L.; Corbett, R. M.; Hirschmann, R. Org. Lett. 2000, 2, 3887–3890. doi:10.1021/ol0066330 |
29. | Coutrot, P.; Grison, C.; Charbonnier-Gérardin, C. Tetrahedron 1992, 48, 9841–9868. doi:10.1016/S0040-4020(01)92278-1 |
30. | McKenna, C. E.; Higa, M. T.; Cheung, N. H.; McKenna, M.-C. Tetrahedron Lett. 1977, 18, 155–158. doi:10.1016/S0040-4039(01)92575-4 |
7. | Wrona-Piotrowicz, A.; Zakrzewski, J.; Métivier, R.; Brosseau, A.; Makal, A.; Woźniak, K. RSC Adv. 2014, 4, 56003–56012. doi:10.1039/C4RA07045C |
31. | Goldberg, J. M.; Wissner, R. F.; Klein, A. M.; Petersson, E. J. Chem. Commun. 2012, 48, 1550–1552. doi:10.1039/c1cc14708k |
7. | Wrona-Piotrowicz, A.; Zakrzewski, J.; Métivier, R.; Brosseau, A.; Makal, A.; Woźniak, K. RSC Adv. 2014, 4, 56003–56012. doi:10.1039/C4RA07045C |
21. | Niko, Y.; Cho, Y.; Kawauchi, S.; Konishi, G.-i. RSC Adv. 2014, 4, 36480–36484. doi:10.1039/C4RA06282E |
22. | Niko, Y.; Konishi, G.-i. Macromolecules 2012, 45, 2327–2337. doi:10.1021/ma3001252 |
23. | Niko, Y.; Hiroshige, Y.; Kawauchi, S.; Konishi, G.-i. J. Org. Chem. 2012, 77, 3986–3996. doi:10.1021/jo300317r |
24. | Niko, Y.; Hiroshige, Y.; Kawauchi, S.; Konishi, G.-i. Tetrahedron 2012, 68, 6177–6185. doi:10.1016/j.tet.2012.05.072 |
25. | Hwang, J.; Choi, M. G.; Eor, S.; Chang, S.-K. Inorg. Chem. 2012, 51, 1634–1639. doi:10.1021/ic2019428 |
26. | Kerr, C. E.; Mitchell, C. D.; Ying, Y.-M.; Eaton, B. E.; Netzel, T. L. J. Phys. Chem. B 2000, 104, 2166–2175. doi:10.1021/jp993776z |
27. | Kerr, C. E.; Mitchell, C. D.; Headrick, J.; Eaton, B. E.; Netzel, T. L. J. Phys. Chem. B 2000, 104, 1637–1650. doi:10.1021/jp992773j |
28. | Frazer, J. D.; Horner, S. M.; Woski, S. A. Tetrahedron Lett. 1998, 39, 1279–1282. doi:10.1016/S0040-4039(97)10853-X |
7. | Wrona-Piotrowicz, A.; Zakrzewski, J.; Métivier, R.; Brosseau, A.; Makal, A.; Woźniak, K. RSC Adv. 2014, 4, 56003–56012. doi:10.1039/C4RA07045C |
7. | Wrona-Piotrowicz, A.; Zakrzewski, J.; Métivier, R.; Brosseau, A.; Makal, A.; Woźniak, K. RSC Adv. 2014, 4, 56003–56012. doi:10.1039/C4RA07045C |
© 2015 Wrona-Piotrowicz et al; licensee Beilstein-Institut.
This is an Open Access article under the terms of the Creative Commons Attribution License (http://creativecommons.org/licenses/by/2.0), which permits unrestricted use, distribution, and reproduction in any medium, provided the original work is properly cited.
The license is subject to the Beilstein Journal of Organic Chemistry terms and conditions: (http://www.beilstein-journals.org/bjoc)