Abstract
Biphenyl-2,2’-bisfenchyloxydichlorosilane (7, BIFOXSiCl2) is synthesized and employed as precursor for the new silanols biphenyl-2,2’-bisfenchyloxychlorosilanol (8, BIFOXSiCl(OH)) and biphenyl-2,2’-bisfenchyloxysilanediol (9, BIFOXSi(OH)2). BIFOXSiCl2 (7) shows a remarkable stability against hydrolysis, yielding silanediol 9 under enforced conditions. A kinetic study for the hydrolysis of dichlorosilane 7 shows a 263 times slower reaction compared to reference bis-(2,4,6-tri-tert-butylphenoxy)dichlorosilane (14), known for its low hydrolytic reactivity. Computational analyses explain the slow hydrolyses of BIFOXSiCl2 (7) to BIFOXSiCl(OH) (8, Ea = 32.6 kcal mol−1) and BIFOXSiCl(OH) (8) to BIFOXSi(OH)2 (9, Ea = 31.4 kcal mol−1) with high activation barriers, enforced by endo fenchone units. Crystal structure analyses of silanediol 9 with acetone show shorter hydrogen bonds between the Si–OH groups and the oxygen of the bound acetone (OH···O 1.88(3)–2.05(2) Å) than with chlorosilanol 8 (OH···2.16(0) Å). Due to its two hydroxy units, the silanediol 9 shows higher catalytic activity as hydrogen bond donor than chlorosilanol 8, e.g., C–C coupling N-acyl Mannich reaction of silyl ketene acetals 11 with N-acylisoquinolinium ions (up to 85% yield and 12% ee), reaction of 1-chloroisochroman (18) and silyl ketene acetals 11 (up to 85% yield and 5% ee), reaction of chromen-4-one (20) and silyl ketene acetals 11 (up to 98% yield and 4% ee).
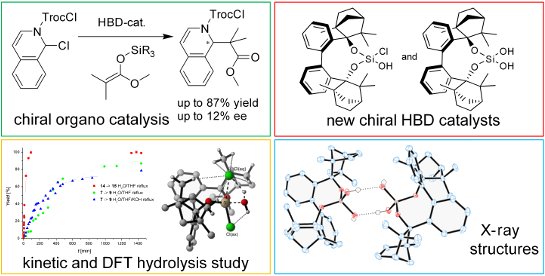
Graphical Abstract
Introduction
Silanediols are attractive target molecules due their hydrogen-bonding capabilities [1-6]. Two synthetic routes are available for syntheses of organosilanediols: If diphenylsilanes are used as building blocks, this route is well suited for syntheses of silanediols with electrophilic functions. In this case, the phenyl groups at the silicon atom are converted by acids (e.g., TFA or TfOH) and following aqueous work-up into silanediols [2,4,7-11]. Another route employs dichlorosilanes, which hydrolyze directly with nucleophiles (e.g., water [12-17] or hydroxide [18-22]) to the corresponding silanediols. While hydrolyses of dichlorosilanes have been studied extensively [23-25], hydrolyses of alkoxy dichlorosilanes are much less explored.
Hydrogen bond donor (HBD) catalysis is an emerging field in organic synthesis [26-28], employing, e.g., squaramides [29], (thio)ureas [30,31] and phosphoric acid derivatives [27]. Cyclodiphosph(V)azanes [32-35] and silanediols [1,28,36] are two new hydrogen bonding scaffolds for anion recognition [37] and ion-pair catalysis [6,38]. Since Kondo et al. established silanediol 1 [39] as HBD for anion recognition in 2006, new chiral and achiral silanediols with organosilicon units have been developed by the groups of Franz (2) [40-44] and Mattson (3, 4) [45-49] (Figure 1, Scheme 1). While these new catalysts have been proven to be potent HBD catalysts, the syntheses are challenging [47]. Compared to these stable carbon-connected silanediols, the readily accessible alkoxy silanediols undergo fast condensation reactions which often lead to unknown and insoluble polysiloxanes [50,51].
Figure 1: Hydrogen-bonding silanediols, i.e., di(1-naphthyl)silanediol (1) [39], silanediols 2 [41-43], binaphthylsilanediol derivatives 3 [45,46] and 4 [47,48] and novel biphenyl-2,2’-bisfenchyloxydichlorosilane (7), biphenyl-2,2’-bisfenchyloxychlorohydroxysilane (8) and biphenyl-2,2’-bisfenchyloxysilanediol (9) with precursor BIFOL (5) [52] and phosphite derivative BIFOP-Cl (6) [53].
Figure 1: Hydrogen-bonding silanediols, i.e., di(1-naphthyl)silanediol (1) [39], silanediols 2 [41-43], binaphthylsilane...
Scheme 1: Hydrogen-bond-catalyzed N-acyl Mannich reaction of in situ-generated isoquinolin derivative 10 with different silyl ketene acetals 11 yielding C–C coupling product 12 [45,47].
Scheme 1: Hydrogen-bond-catalyzed N-acyl Mannich reaction of in situ-generated isoquinolin derivative 10 with...
Previously, our group reports syntheses and applications of symmetric biphenyl-2,2`-bisfenchol (5, BIFOL, Figure 1) [52,54] and it´s derivative, the chiral chlorophosphite ligand 6 (BIFOP-Cl, Figure 1), e.g., in Cu-catalyzed 1,4-additions [53], in Pd-catalyzed alkyl–aryl cross coupling reactions [55,56], as well as for organoaluminum fencholate reagents [57]. Unexpected stability against hydrolysis [58] makes BIFOL (5) a potentially promising chiral backbone for new organo silicates, e.g., silanediol 9 (Figure 1). As a silicic acid ester, silanediol 9 should show increased acidity in comparison to C–Si(OH)2 derivates, e.g., 1–4 [40]. In this work the syntheses of BIFOXSiCl2 (7), BIFOXSi(OH)2 (9) and BIFOXSiCl(OH) (8) are described. The hydrolytic stability of dichlorosilane 7 is investigated in a kinetics study and is compared to analogue dichlorosilanes, i.e., 13 and 14 (Scheme 4). UV–vis titration experiments and catalyses are carried out with chlorosilanol 8 and silanediol 9, to assess catalytic and anion binding characteristics.
Results and Discussion
Enantiopure dichlorosilane 7 is readily accessible by lithiation of BIFOL (5) [28,52,54,59] and subsequent reaction with tetrachlorosilane (92% yield, Scheme 2).
Scheme 2: Synthesis of BIFOXSiCl2, starting with BIFOL (5) [52,54] yielding dichlorosilane 7.
Scheme 2: Synthesis of BIFOXSiCl2, starting with BIFOL (5) [52,54] yielding dichlorosilane 7.
Unlike the hydrolysis of BIFOP-Cl (6) to BIFOP-OH, the dichlorosilane 7 is not hydrolyzed by aqueous potassium hydroxide solution [53]. The heterolytic reaction of solid BIFOXSiCl2 (7) in an aqueous KOH solution is negligible (<1% yield, Table 1, Scheme 3 in a) H2O and b) H2O/KOH). The reluctance against hydrolysis of BIFOXSiCl2 (7) can be explained by the hydrophobic aryl backbone and the fenchyl groups, which result in a decrease of the solubility of BIFOXSiCl2 (7) in water. Thus, a H2O/THF mixture is used to increase solubility and yields (Table 1, Scheme 3c). While the solubility of BIFOXSiCl2 (7) in H2O/THF greatly increases (clear solution), potassium hydroxide is needed as a strong nucleophile to yield BIFOXSi(OH)2 (9) at 20 °C (14% yield, Table 1, Scheme 3d). By increasing the temperature to 50 °C the hydrolysis increases, resulting in 32% yield in H2O/THF and 64% yield in H2O/THF/KOH (Table 1, Scheme 3).
Scheme 3: Hydrolysis of BIFOXSiCl2 (7) yielding the corresponding silanediol 9 and controlled hydrolysis of BIFOXSiCl2 (7) to BIFOXSiCl(OH) (8). For solvent mixtures and temperatures see Table 1.
Scheme 3: Hydrolysis of BIFOXSiCl2 (7) yielding the corresponding silanediol 9 and controlled hydrolysis of B...
Table 1: Hydrolysis of BIFOXSiCl2 (7) to BIFOXSi(OH)2 (9) (Scheme 3) in different solvent mixtures, with or without KOH at different temperatures.
yielda [%] in solvent: | ||||
---|---|---|---|---|
a) | b) | c) | d) | |
T | H2O | H2O/KOH | H2O/THF | H2O/THF/KOH |
20 °C | <1 | <1 | <1 | 14 |
50 °C | <1 | <1 | 32 | 64 |
refluxb | <1 | <1 | 84 | 53 |
aIsolated yields, reaction conditions: 0.09 mmol 7, 2.5 mL solvent, 0.9 mmol KOH, H2O/THF 1:1. breflux conditions are: H2O ≈ 100 °C, H2O/KOH ≈ 107 °C, H2O/THF ≈ 77 °C, H2O/THF/KOH ≈ 80 °C.
At reflux conditions in H2O/THF, but without potassium hydroxide, BIFOXSi(OH)2 (9) is isolated in 89% yield, while with KOH, just 53% yield is achieved (Table 1). The lower yield of silanediol 9, at H2O/THF/KOH reflux conditions, can be explained by the further hydrolysis to BIFOL (5, Scheme 3). Under the conditions described in Table 1, the monohydroxy compound BIFOXSiCl(OH) (8) cannot be isolated as an intermediate. For the synthesis of chlorosilanol 8 optimized reaction conditions are necessary. Here, 1 equiv of water and 2 equiv of triethylamine (relative to dichlorosilane 7) are added to a THF solution at 20 °C. BIFOXSiCl(OH) (8) is isolated in 67% yield (Scheme 3).
Hydrolysis studies
To assess the stability of dichlorosilane 7, its hydrolysis relative to established silanediol motifs, i.e., di-tert-butoxydichlorosilane (((CH3)3CO)2SiCl2) [50], di(1-naphthyl)dichlorosilane (13) [39] and bis(2,4,6-tri-tert-butylphenoxy)dichlorosilane (14) [60,61] is examined (Scheme 4). In close analogy to BIFOXSiCl2 (7), di-tert-butoxydichlorosilane is substituted with tertiary alkoxy groups. While the close analogy of BIFOXSiCl2 (7) and ((CH3)3CO)2SiCl2 would make a comparison of these two dichlorosilanes preferable, the instability of the latter against hydrolyses and temperature resulting in further condensation products [50,51], leaves no comparison possible.
Scheme 4: Hydrolysis of dichlorosilanes 13 and 14 to their corresponding silanediols 1 and 15 [51,60].
Scheme 4: Hydrolysis of dichlorosilanes 13 and 14 to their corresponding silanediols 1 and 15 [51,60].
At H2O/THF reflux conditions, the hydrolysis of dichlorosilane 13 yields silanediol 1 with >99% yield, after a reaction time of two seconds (Scheme 4, addition of H2O, with instant extraction with Et2O). The stability of dichlorosilane 14, which has been previously reported by Spirk et al. [60], has been found to be higher than that of dichlorosilane 13 under the same conditions (H2O/THF reflux) resulting in >99% yield after 100 min (Figure 2, red squares). The hydrolysis of dichlorosilanes 7 and 14 to the corresponding silanediols 9 (Scheme 3) and 15 (Scheme 4) is investigated further (Figure 2). The conversion of dichlorosilane 14 to silanediol 15 is completed (yield >99%) after 100 minutes at H2O/THF reflux conditions (Figure 2, red square). The hydrolysis of BIFOXSiCl2 (7) on the other hand is slower at the same conditions (24 h for 84% yield of BIFOXSi(OH)2 (9, Figure 2, green circle). With H2O/THF/KOH reflux conditions the initial reaction is found to be slightly faster, but resulting in a saturation at a lower yield of BIFOXSi(OH)2 (9, Figure 2, blue triangle, 70% yield), caused by a starting decomposition of BIFOXSi(OH)2 (9) to BIFOL (5) and Si(OH)4 (Scheme 3). To quantify the reactivity of the dichlorosilanes 7 and 14, a higher concentration of water (solvent) or hydroxide (10 equiv) is used (Table 2), resulting in a pseudo first order reaction.
![[1860-5397-15-17-2]](/bjoc/content/figures/1860-5397-15-17-2.png?scale=2.0&max-width=1024&background=FFFFFF)
Figure 2: Hydrolyses of dichlorosilane 7 and 14 to BIFOXSi(OH)2 (9, green circle) and bis(2,4,6-tri-tert-butylphenoxy)silanediol (15, red square) in H2O/THF reflux and BIFOXSi(OH)2 (9, blue triangle) in H2O/THF/KOH reflux conditions. Isolated yields are plotted against reaction time (Table 1, Scheme 3 and Scheme 4). Reflux conditions are: H2O/THF ≈ 77 °C, H2O/THF/KOH ≈ 80 °C.
Figure 2: Hydrolyses of dichlorosilane 7 and 14 to BIFOXSi(OH)2 (9, green circle) and bis(2,4,6-tri-tert-buty...
Table 2: Hydrolyses of dichlorosilanes 7 and 14 to the corresponding silanediols 9 and 15 (Figure 3, Scheme 3 and Scheme 4, absolute reaction constant k and relative reaction constant krel).
reaction | ka [min−1] | krelb [min−1] |
---|---|---|
14 H2O/THF refluxc | 0.848 | 263 |
7 H2O/THF/KOH refluxc | 0.005 | 1.5 |
7 H2O/THF refluxc | 0.003 | 1 |
ak is determined with ln ([A0]/[At]) = k * t and plotted in Figure 3. bkrel is normalized on the slowest hydrolysis reaction of BIFOXSiCl2 (7) in H2O/THF reflux conditions (Scheme 2, Scheme 3). cReflux conditions are: H2O/THF ≈ 77 °C, H2O/THF/KOH ≈ 80 °C.
![[1860-5397-15-17-3]](/bjoc/content/figures/1860-5397-15-17-3.png?scale=2.0&max-width=1024&background=FFFFFF)
Figure 3: Hydrolyses of BIFOXSiCl2 (7) to BIFOXSi(OH)2 (9, green circle), bis(2,4,6-tri-tert-butylphenoxy)dichlorosilane (14) to bis(2,4,6-tri-tert-butylphenoxy)silanediol (15, red square) in H2O/THF reflux and BIFOXSiCl2 (7) to BIFOXSi(OH)2 (9, blue triangle) H2O/THF/KOH reflux conditions (Scheme 3 and Scheme 4). Reaction constants are evaluated with ln ([A0]/[At]) = k·t for a pseudo-first-order reaction plotted against reaction time (Table 2). Reflux conditions are: H2O/THF ≈ 77 °C, H2O/THF/KOH ≈ 80 °C.
Figure 3: Hydrolyses of BIFOXSiCl2 (7) to BIFOXSi(OH)2 (9, green circle), bis(2,4,6-tri-tert-butylphenoxy)dic...
To remove the influence of the decomposition of silanediol 9, just the reaction time from 0 to 600 min is considered. Compared to established dichlorosilanes, the observed stability of BIFOXSiCl2 (7) is clearly apparent from those studies (Figure 2 and Figure 3). Dichlorosilane 14, which is known to show a comparably high resistancy against hydrolysis [60], exhibits a much faster hydrolysis reaction (krel = 263 min−1, Table 2) at H2O/THF reflux conditions than BIFOXSiCl2 (7). With KOH, the reaction rate of the hydrolysis of BIFOXSiCl2 (7) is just slightly increased (krel = 1.5 min−1, Table 2).
Computational analyses
Nucleophilic substitution at silicon is already discussed with SN2 mechanism, following a backside attack opposite of the leaving group, as well as a front side attack near the leaving group [62-68]. A backside attack at the silicon in dichlorosilane 7 and monochlorosilanol 8 is blocked by the backbone, making a consideration of the mechanism not necessary. A mechanism with a pentacoordination at the silicon is assumed for the hydrolyses of BIFOXSiCl2 (7) to BIFOXSiCl(OH) (8) as intermediate and BIFOXSI(OH)2 (9) as product [65-67]. Two pathways (front attack mechanism (front) or side attack mechanism (side)) for the approaching water molecule are considered (Scheme 5).
Scheme 5: Two investigated pathways for the hydrolysis of the dichlorosilanes. Front attack mechanism (front) between the two chloro substituents or side attack mechanism (side) between one chloro and both additional substituents (TS = transition structure) [62-68].
Scheme 5: Two investigated pathways for the hydrolysis of the dichlorosilanes. Front attack mechanism (front)...
In both, front attack and side attack, the attacking water molecule is in plane with the Cl–Si–Cl unit for the first hydrolysis step. For the second hydrolysis step, analogue pathways are considered. These trajectories lead to three transition structures each, for the hydrolysis of BIFOXSiCl2 (7) and BIFOXSiCl(OH) (8, Figure 4).
![[1860-5397-15-17-4]](/bjoc/content/figures/1860-5397-15-17-4.png?scale=2.0&max-width=1024&background=FFFFFF)
Figure 4: Three transition structures each, for the hydrolysis of BIFOXSiCl2 (7) and BIFOXSiCl(OH) (8) considering two possible configuration isomers of BIFOXSiCl(OH) (8). For 8ax the OH group is parallel situated to the biaryl axis. For 8eq the OH group is orthogonal oriented to the biaryl axis. The fenchyl groups are abbreviated with (*) for more clarity.
Figure 4: Three transition structures each, for the hydrolysis of BIFOXSiCl2 (7) and BIFOXSiCl(OH) (8) consid...
Geometry optimizations and frequency computations are performed in gas phase with B3LYP-D3BJ/6-31G(d) at 298 K. For single point energies, M06-2X-D3/6-311++G(d,p) in the solvent THF with the PCM model is used [69,70]. The free Gibbs energies of the respective structures are discussed. The activation energy (Ea) is the difference between the educt and the TS and the reaction energy (Er) is the difference between the educts and products of the respective steps. The mechanism of hydrolysis, only one molecule of water per hydrolysis step is considered. Additional interactions by THF and water are only considered by the PCM model. Starting with BIFOXSiCl2 (7), the side and front1 attack mechanism are resulting in BIFOXSiCl(OH) 8eq. The front2 attack mechanism results in BIFOXSiCl(OH) 8ax (Figure 5).
![[1860-5397-15-17-5]](/bjoc/content/figures/1860-5397-15-17-5.png?scale=2.0&max-width=1024&background=FFFFFF)
Figure 5: Computed hydrolyses of BIFOXSiCl2 (7) to BIFOXSiCl(OH) 8ax and BIFOXSiCl(OH) 8eq and subsequent computed hydrolysis to BIFOXSi(OH)2 (9) and comparison with glycoxydichlorosilane. The activation energy (Ea) is the difference of the free Gibbs energy of the educt and the TS and the reaction energy (Er) is the difference the free Gibbs energies of the educts and products of the respective steps side and front1 resulting in BIFOXSiCl(OH) 8eq, front2 results in BIFOXSiCl(OH) 8ax. From BIFOXSiCl(OH) 8eq only front2 is a possible path to BIFOXSi(OH)2 (9). From BIFOXSiCl(OH) 8ax front1 and side are possible paths to BIFOXSi(OH)2 (9, Table 3, Figure 4, Scheme 5). Reaction energies are (Er) in kcal mol−1, activation energies are (Ea) in kcal mol−1 and italic.
Figure 5: Computed hydrolyses of BIFOXSiCl2 (7) to BIFOXSiCl(OH) 8ax and BIFOXSiCl(OH) 8eq and subsequent com...
For the TS of the front1 attack mechanism, the lowest activation energy (Ea = 32.6 kcal mol−1, Table 3, entry 1, Figure 5 and Figure 6) is found, closely followed by the front2 attack mechanism (Ea = 33.2 kcal mol−1, Table 3, entry 2, Figure 5 and Figure 7). The side attack mechanism leads to the highest TS for the first hydrolysis step of BIFOXSiCl2 (7) to BIFOXSiCl(OH) 8eq (Ea = 37.3 kcal mol−1, Table 3, entry 3, Figure 5 and Figure 8). BIFOXSiCl(OH) 8ax is found to be the more stable isomer with a reaction energy Er of −4.4 kcal mol−1, compared to BIFOXSiCl(OH) 8eq with a Er of −1.7 kcal mol−1 (ΔEr = 2.7 kcal mol−1, Table 3, entries 1 and 2, Figure 5), as it is found as the only isomere in crystal structure analysis (Figure 13). For the second hydrolysis step from BIFOXSiCl(OH) 8ax to BIFOXSi(OH)2 (9), the side attack mechanism leads to the lowest TS (Ea = 31.4 kcal mol−1, Table 3, entry 6, Figure 5, Figure 9), followed by the front1 attack mechanism (Ea = 33.4 kcal mol−1, Table 3, entry 4, Figure 5, Figure 10) leading to product BIFOXSi(OH)2 (9).
Table 3: Computeda activation energies and reaction energies (Ea and Er [kcal mol−1]) and imaginary frequencies (ν [cm−1]) of the transition structure (TS) for the hydrolysis of dichlorosilane 7, 8, 13, [CH2O]2SiCl2 and SiCl4 to the corresponding mono- and diols.
entry | reaction | TS | ν | Ea | Er |
---|---|---|---|---|---|
1b | 7 to 8eq | TSfront1 7 | –206.23 | 32.6 | −1.7 |
2 | 7 to 8ax | TSfront2 7 | –221.44 | 33.2 | −4.4 |
3 | 7 to 8eq | TSside 7 | –189.66 | 37.3 | −1.7 |
4 | 8ax to 9 | TSfront1 8ax | –208.01 | 33.4 | −5.3 |
5 | 8eq to 9 | TSfront2 8eq | –242.41 | 40.2 | −5.3 |
6b | 8ax to 9 | TSside 8ax | –162.28 | 31.4 | −5.3 |
7b | 13 to 13ClOH | TSfront 13 | –167.52 | 27.7 | −1.1 |
8 | 13 to 13ClOH | TSside 13 | –176.02 | 35.4 | −1.1 |
9b | 13ClOH to 1 | TSfront 13ClOH | –137.30 | 29.1 | 1.5 |
10 | 13ClOH to 1 | TSside 13ClOH | −119.75 | 29.9 | 1.5 |
11 | [CH2O]2SiCl2 to [CH2O]2SiClOH | –542.44 | 23.6 | −4.0 | |
12 | [CH2O]2SiClOH to [CH2O]2Si(OH)2 | –189.64 | 21.1 | −4.7 | |
13 | Cl2SiCl2 to Cl2SiClOH | –415.78 | 28.4 | −5.4 | |
14 | Cl2SiClOH to Cl2Si(OH)2 | –209.82 | 25.0 | −9.3 | |
15 | (OH)2SiCl2 to (OH)2SiClOH | –145.49 | 20.9 | −3.4 | |
16 | (OH)2SiClOH to (OH)2Si(OH)2 | –128.55 | 20.8 | −4.8 |
aM06-2X-D3/6-311++G(d.p)(PCM=THF)//B3LYP-D3BJ/6-31G(d) at 298 K. bFavored reaction structures are bolted.
From BIFOXSiCl(OH) 8eq only the front attack mechanism TSfront2 8eq is possible, which also leads to BIFOXSi(OH)2 (9), but with the highest Ea (40.2 kcal mol−1, Table 3, entry 5, Figure 5 and Figure 11). In accordance with the crystal structure analysis of BIFOXSiCl(OH) (8, Figure 13), it can be seen that the more stable isomer BIFOXSiCl(OH) 8ax corresponds to the synthesized isomer. Considering the lowest Ea for both steps, the first hydrolysis step is the rate-determining step (7 to 8eq, TSfront1 7 Ea = 32.6 kcal mol−1 vs 8ax to 9, TSside 8ax Ea = 31.4 kcal mol−1, Table 3, entries 1 and 6, Figure 6 and Figure 9), which agrees with the experimental hydrolysis. Under H2O/THF reflux conditions, no BIFOXSiCl(OH) (8) has been isolated, but has to be synthesized separately (Scheme 3, Figure 2 and Figure 3). Both front attack TS have much lower energy, than the TS resulting by side attack mechanism, for the first hydrolysis step (TSfront2 7 Ea = 33.2 kcal mol−1, TSfront1 7 Ea = 32.6 kcal mol−1 vs TSside 7 Ea = 37.3 kcal mol−1, Table 3, entries 1–3, Figures 6–8). Responsible for the lower Ea is an additional stabilization by an interaction of the remaining chloro atom to the attacking water (dotted line to the Cl(ax) Figure 6 and Cl(eq) Figure 7). The small energy difference for the TSfront1 7 and TSfront2 7 is to explained by additional C–H interactions between the fenchyl groups to the leaving chloride (four dotted lines in TSfront2 7, Figure 7, five dotted lines in TSfront1 7, Figure 6).
![[1860-5397-15-17-6]](/bjoc/content/figures/1860-5397-15-17-6.png?scale=2.0&max-width=1024&background=FFFFFF)
Figure 6: Transition state leading to 8eq following front1 attack (Ea = 32.6 kcal mol−1, Figure 5, Table 3, entry 1). Breaking and forming bonds in dashed lines, additional C–H-interactions with dotted lines (M06-2X-D3/6-311++G(d,p)(PCM=THF)//B3LYP-D3BJ/6-31G(d) at 298 K).
Figure 6: Transition state leading to 8eq following front1 attack (Ea = 32.6 kcal mol−1, Figure 5, Table 3, entry 1). Breaki...
![[1860-5397-15-17-7]](/bjoc/content/figures/1860-5397-15-17-7.png?scale=2.0&max-width=1024&background=FFFFFF)
Figure 7: Transition state leading to 8ax following front2 attack (Ea = 33.2 kcal mol−1, Figure 5, Table 3, entry 2). Breaking and forming bonds in dashed lines, additional C–H-interactions with dotted lines (M06-2X-D3/6-311++G(d,p)(PCM=THF)//B3LYP-D3BJ/6-31G(d) at 298 K).
Figure 7: Transition state leading to 8ax following front2 attack (Ea = 33.2 kcal mol−1, Figure 5, Table 3, entry 2). Breaki...
Through the approach of the attacking water molecule in the side attack mechanism, the chloro atoms are forced to get closer to each other leading to electrostatic repulsion (Figure 8). Stabilizing C–H interaction from the fenchyl group to the exiting chloride can be found as well (one dotted line in TSside 7, Figure 8).
![[1860-5397-15-17-8]](/bjoc/content/figures/1860-5397-15-17-8.png?scale=2.0&max-width=1024&background=FFFFFF)
Figure 8: Transition state leading to 8eq following side attack (Ea = 37.4 kcal mol−1, Figure 5, Table 3, entry 3). Breaking and forming bonds in dashed lines, additional C–H-interactions with dotted lines (M06-2X-D3/6-311++G(d,p)(PCM=THF)//B3LYP-D3BJ/6-31G(d) at 298 K).
Figure 8: Transition state leading to 8eq following side attack (Ea = 37.4 kcal mol−1, Figure 5, Table 3, entry 3). Breaking...
At the second step, the side mechanism leads to a lower energy barrier (TSside 8ax Ea = 31.4 kcal mol−1, Table 3, entry 6, Figure 9) than the front attack mechanisms (TSfront1 8ax Ea = 33.4 kcal mol−1, TSfront2 8eq Ea = 40.2 kcal mol−1, Table 3, entries 4 and 5, Figure 10 and Figure 11). In the former mechanism the chloro atom comes closer to the already present hydroxy group (Figure 9).
![[1860-5397-15-17-9]](/bjoc/content/figures/1860-5397-15-17-9.png?scale=2.0&max-width=1024&background=FFFFFF)
Figure 9: Transition state leading to 9 following side attack (Ea = 31.4 kcal mol−1, Figure 5, Table 3, entry 6). Breaking and forming bonds in dashed lines, additional C–H-interactions with dotted lines (M06-2X-D3/6-311++G(d,p)(PCM=THF)//B3LYP-D3BJ/6-31G(d) at 298 K).
Figure 9: Transition state leading to 9 following side attack (Ea = 31.4 kcal mol−1, Figure 5, Table 3, entry 6). Breaking a...
A contact between the OH(ax) and the Cl(eq) is found, in addition to the C–H interaction (dotted line, Figure 9), which stabilized the leaving Cl ion with a weak hydrogen bond. In the front attack mechanisms for the second hydrolytic step only stabilizing C–H interactions from the fenchyl group to the chloro atom occur (dotted line, Figure 10 and Figure 11).
![[1860-5397-15-17-10]](/bjoc/content/figures/1860-5397-15-17-10.png?scale=2.0&max-width=1024&background=FFFFFF)
Figure 10: Transition state leading to 9 following front1 attack (Ea = 33.4 kcal mol−1, Figure 5, Table 3, entry 4). Breaking and forming bonds in dashed lines, additional C–H-interactions with dotted lines (M06-2X-D3/6-311++G(d,p)(PCM=THF)//B3LYP-D3BJ/6-31G(d) at 298 K).
Figure 10: Transition state leading to 9 following front1 attack (Ea = 33.4 kcal mol−1, Figure 5, Table 3, entry 4). Breaking...
![[1860-5397-15-17-11]](/bjoc/content/figures/1860-5397-15-17-11.png?scale=2.0&max-width=1024&background=FFFFFF)
Figure 11: Transition state leading to 9 following front2 attack (Ea = 40.2 kcal mol−1, Figure 5, Table 3, entry 5). Breaking and forming bonds in dashed lines, additional C–H-interactions with dotted lines (M06-2X-D3/6-311++G(d,p)(PCM=THF)//B3LYP-D3BJ/6-31G(d) at 298 K).
Figure 11: Transition state leading to 9 following front2 attack (Ea = 40.2 kcal mol−1, Figure 5, Table 3, entry 5). Breaking...
The highest energy barriers of the computed molecules are found for BIFOXSiCl2 for the first step (Ea = 32.6 kcal mol−1, Ea = 33.2 kcal mol−1, Ea = 37.3 kcal mol−1, Table 3, entries 1–3, Figures 6–8) and for BIFOXSiCl(OH) (8) for the second step (Ea = 33.4 kcal mol−1, Ea = 31.4 kcal mol−1, Ea = 40.2 kcal mol−1, Table 3, entries 4–6, Figures 9–11), which also confirms the kinetics study of BIFOXSiCl2 (7) and BIFOXSiCl(OH) (8) as the most stable dichlorosilane and chlorosilanol (Figure 2 and Figure 3, Table 2). In comparison the front attack mechanism for dichlorosilane 13 (Ea = 27.7 kcal mol−1, Table 3, entry 7) has a lower energy barrier than side attack mechanism (Ea = 35.4 kcal mol−1, Table 3, entry 8). The second hydrolysis, 13ClOH to 1 has a higher energy barrier (Ea = 29.1 kcal mol−1 for front attack mechanism (Table 3, entry 9) and Ea = 29.9 kcal mol−1 for side attack mechanism (Table 3, entry 10)) than the first. In accordance with the kinetic study, dichlorosilane 13 hydrolysed faster than BIFOXSiCl2 (7, Scheme 4). In addition, the hydrolysis of glycol-based dichlorosilane (Table 3, entries 11 and 12) and tetrachlorosilane (Table 3, entries 13 to 16) is computed as model system. The first hydrolytic step of SiCl4 has a higher energy barrier (Ea = 28.4 kcal mol−1, Table 3, entry 13) than 13 to 13ClOH (Ea = 27.7 kcal mol−1, Table 3, entry 7) and 13ClOH to 1 (Ea = 29.1 kcal mol−1, Table 3, entry 9). The glycol-substituted dichlorosilane has a smaller energy barrier to the TS (Ea = 23.6 kcal mol−1, Table 3, entry 11, Figure 5 and Ea = 21.1 kcal mol−1, Table 3, entry 12, Figure 5). The stepwise hydrolysis of tetrachlorosilane shows that the energy barrier for the first step is higher (Ea = 28.4 kcal mol−1, Table 3, entry 13), than the second step (Ea = 25.0 kcal mol−1, Table 3, entry 14). With two hydroxy substituents, the energy barrier for the third TS is Ea = 20.9 kcal mol−1 (Table 3, entry 15) and for the fourth step (Ea = 20.8 kcal mol−1, Table 3, entry 16). For the computed values, it should be noted that THF is used for the PCM solvent correction, but the reactions are carried out in a 1/1 mixture of water/THF.
A hydrogen bridge is a displacement of electrons (lp or π) from a donor into the σ* orbital of an H–X bond. NBO analyses can be used to calculate the energy of such an interaction. With the shift of the electron density of a lone pair into the σ* orbital, the O–H bond of the HB donor is weakened. This causes a change in the O–H stretching frequency of the bond. A strong hydrogen bridge results from strong lone pair···σ* orbital interactions, resulting in a weakening of the O–H bond, which in turn results in a decrease of the O–H stretching frequency [71]. An alternative method is to determine the relaxed force constant [72]. In the following, the stretching frequency und the NBO analyses were calculated for different acceptors and water (Table 4).
Table 4: Computeda stretching frequencies (ν [cm−1]), lp···σ* [kcal mol−1] and donor acceptor distances (D [Å]) of silanes 7, 8, [CH2O]2SiCl(OH), [CH2O]2Si(OH)2, (OH)2SiCl(OH), Si(OH)4, methanol and [CH2O]2C(OH)2.
entry |
νO-H
[cm−1] |
lp-σ* energy
[kcal mol−1] |
D O···X
[Å] |
|
---|---|---|---|---|
1 | CH3-OH···OH2 | 3741 | 11.88 | 2.785 |
2 | SiH3-OH···OH2 | 3602 | 16.43 | 2.793 |
3 |
Si(OH)3-OH···OH2
H2O···OH-Si(OH)3 |
3441
3608 |
20.39
12.05 |
2.732
2.797 |
4 |
SiCl(OH)2-OH···OH2
H2O···OH-SiCl(OH)2 |
3450
3649 |
19.44
7.78 |
2.727
2.862 |
5 |
SiCl(OH)2-OH···OH2
SiCl(OH)2-OH···OH2 H2O···Cl-Si(OH)3 |
3725
3701 3832 |
6.78
6.82 1.58 |
2.865
2.863 2.804 |
6 | (CH2)2O2Si-(OH)2···OH2 |
3745b
3717b 3691c |
7.62
7.59 |
2.855
2.857 |
7 |
(CH2)2O2SiCl-OH···OH2
H2O···Cl-Si(OH)O2(CH2)2 |
3377
3819c 3707b |
22.16
1.58 |
2.705
3.331 |
8 |
(CH2)2O2C-(OH)2···OH2
(CH2)2O2C-(OH)2···OH2 H2O···O2(CH2)2C(OH)2 |
3678c
3631c 3604c |
6.57
8.69 8.96 |
2.844
2.816 2.715 |
9 |
BIFOXSiCl-OH···OH2
BIFOXSi(OH)-Cl···H2O |
3445
3691b 3811c |
24.90
5.19 |
2.734
3.273 |
10 | BIFOXSi(OH)2···OH2 |
3733b
3703c 3685c |
9.73
10.15 |
2.878
2.834 |
11 | BIFOXSiCl-OH···Cl- | 3165 | 36.87 | 2.994 |
12 | BIFOXSi(OH)2···Cl- |
3421
3151 |
17.36
33.61 |
3.082
2.927 |
13 | BIFOXSi(OH)2 – dimer |
3670
3490 |
7.55
21.63 |
2.880
2.752 |
aComputed with B3LYP-D3BJ/6-31G(d) at 298 K; bsymmetric stretching frequency νs; casymmetric stretching frequency νas.
The silanol group is a better hydrogen bond acceptor than an alcohol group for single hydrogen bonds (CH3OH (11.88 kcal mol−1) vs SiH3OH (16.43 kcal mol−1), Table 4, entries 1 and 2), which is more acidic and inconsistent with the results of West et al. [73]. In case of double hydrogen bonds in the glyoxal based system, both are equally strong, because of a third hydrogen bond, a rebond from where water is the acceptor and the oxygen in the ring is the donor ((CH2)2O2Si(OH)2 (15.21 kcal mol−1) vs (CH2)2O2C(OH)2 (15.26 kcal mol−1), Table 4, entries 6 and 8), Two possible geometries can be observed for SiCl(OH)3. On the one hand with two hydrogen bridges to water (13.60 kcal mol−1, Table 4, entry 5) or with one hydrogen bridge to water (19.44 kcal mol−1, Table 4, entry 4), with an additional rebond of the oxygen of one of the SiOH groups to water H–O (7.78 kcal mol−1, Table 4, entry 4). The trend of the stronger single hydrogen bridge compared to the double hydrogen bridge is also reflected in the systems glycolic (22.16 kcal mol−1 vs 15.21 kcal mol−1, Table 4, entries 6 and 7) and BIFOSi (24.90 kcal mol−1 vs 19.88 kcal mol−1, Table 4, entries 9 and 10). As was to be expected, the energies of the O–H bonds decreases with increasing lone pair···σ* orbital interactions (3502 cm−1 to 22.16 kcal mol−1 vs 3830 cm−1 to 3.06 kcal mol−1, Table 4, entries 5 and 7). With a stronger electron donor, as the chloride ion, the lone pair···σ* orbital interactions rises (36.87 kcal mol−1 vs 50.97 kcal mol−1, Table 4, entries 11 and 12). In here, the BIFOXSi(OH)2 (9) binds the chlorid stronger with two hydrogen bridges, than BIFOXSiCl(OH) (8) with just one hydrogen bridge. The BIFOXSi(OH)2 (9) dimer forms hydrogen bridges, which are stronger than hydrogen bridges with water, but less stronger than hydrogen bridges to chloride (50.97 kcal mol−1 vs 29.18 kcal mol−1 vs 19.88 kcal mol−1, Table 4, entries 13, 12, and 10).
X-ray analyses
Dichlorosilane 7 (Figure 12), as well as chlorosilanol 8 (Figure 13) crystallize as monomers from n-hexane. BIFOXSiCl(OH) (8) is obtained as the 8ax isomer, which is computed to be the more stable isomer (Table 3, entry 1).
![[1860-5397-15-17-12]](/bjoc/content/figures/1860-5397-15-17-12.png?scale=2.0&max-width=1024&background=FFFFFF)
Figure 12: X-ray crystal structure of BIFOXSiCl2 (7). H atoms on the chiral backbone are omitted for clarity issues and the ellipsoids are shown with 65% probability.
Figure 12: X-ray crystal structure of BIFOXSiCl2 (7). H atoms on the chiral backbone are omitted for clarity i...
![[1860-5397-15-17-13]](/bjoc/content/figures/1860-5397-15-17-13.png?scale=2.0&max-width=1024&background=FFFFFF)
Figure 13: X-ray crystal structure of BIFOXSiCl(OH) (8). H atoms on the chiral backbone are omitted for clarity issues and the ellipsoids are shown with 65% probability.
Figure 13: X-ray crystal structure of BIFOXSiCl(OH) (8). H atoms on the chiral backbone are omitted for clarit...
Since the commercially available (+)-fenchone has 98% enantiomeric purity, BIFOXSi(OH)2 can be further purified. rac-BIFOXSi(OH)2 crystallizes as a dimer from toluene (Figure 14). BIFOXSi(OH)2 (9) crystallizes as a tetramer from n-hexane (Figure 15), where six OH groups build a network of hydrogen bonds. Thus the polar core is shielded against the solvent (Figure 15). For silanediols 2a and 2b (Figure 1) dimeric structures are reported as well [41-44]. In these, hydrogen bond lengths of 1.86 Å to 2.01 Å (H···O) and 2.65–2.80 Å (O–H···O) are observed. Bond angles vary from 157.3° to 174.3° for the hydrogen bonds between the silanediols [41-44]. In the dimeric and tetrameric structure of BIFOXSi(OH)2 (9, Figure 14 and Figure 15) those distances are 1.82(4) Å to 2.02(4) Å (H···O) and 2.66(4) Å to 2.79(5) Å (O–H···O) with angles between 152,4(6)° and 172,0(7)° (Table 5). These distances indicate medium strong hydrogen bonds [74].
![[1860-5397-15-17-14]](/bjoc/content/figures/1860-5397-15-17-14.png?scale=2.0&max-width=1024&background=FFFFFF)
Figure 14: X-ray crystal structure ofrac-BIFOXSi(OH)2 (9) forming dimers. H atoms on the chiral backbone are omitted for clarity issues and the ellipsoids are shown with 65% probability. For bond lengths and angles see Table 5.
Figure 14: X-ray crystal structure ofrac-BIFOXSi(OH)2 (9) forming dimers. H atoms on the chiral backbone are o...
![[1860-5397-15-17-15]](/bjoc/content/figures/1860-5397-15-17-15.png?scale=2.0&max-width=1024&background=FFFFFF)
Figure 15: X-ray crystal structure of BIFOXSi(OH)2 (9) forming a tetramer. H atoms on the chiral backbone are omitted for clarity issues and the ellipsoids are shown with 65% probability. For bond lengths and angles see Table 5.
Figure 15: X-ray crystal structure of BIFOXSi(OH)2 (9) forming a tetramer. H atoms on the chiral backbone are ...
X-ray structures of BIFOXSi(OH)2 (9, Figure 16) and BIFOXSiCl(OH) (8, Figure 17) with co-crystallized acetone indicate the bonding behavior of the silanediols to carbonyl acceptors.
![[1860-5397-15-17-16]](/bjoc/content/figures/1860-5397-15-17-16.png?scale=2.0&max-width=1024&background=FFFFFF)
Figure 16: X-ray crystal structure of BIFOXSi(OH)2 (9) forming a dimeric structure with two bridged acetone molecules. H atoms on the chiral backbone are omitted for clarity issues. The acetone on the upper side is disordered by 50%. Ellipsoids are shown with 65% probability. For bond lengths and angles see Table 5.
Figure 16: X-ray crystal structure of BIFOXSi(OH)2 (9) forming a dimeric structure with two bridged acetone mo...
![[1860-5397-15-17-17]](/bjoc/content/figures/1860-5397-15-17-17.png?scale=2.0&max-width=1024&background=FFFFFF)
Figure 17: X-ray crystal structure of BIFOXSiCl(OH) (8), binding an acetone molecule. H atoms on the chiral backbone are omitted for clarity issues and the ellipsoids are shown with 65% probability. For bond lengths and angles see Table 5.
Figure 17: X-ray crystal structure of BIFOXSiCl(OH) (8), binding an acetone molecule. H atoms on the chiral ba...
Chlorosilanol 8 binds one acetone with a bonding length of 2.16(0) Å (H3···O4) and 2.89(4) Å (O3–H···O4) (Table 5, Figure 17), which is the longest hydrogen bond for BIFOXSiCl(OH) (8) and BIFOXSi(OH)2 (9). In dimeric structures of silanediol 9 (Figure 14) the hydroxy group O4H is a hydrogen bond donor to O3. The hydrogen atom of the O–H group (O3H) is pointing outwards and can form a hydrogen bond to an additional molecule. For silanediols 2a and 2b Franz et al. observed hydrogen bond distances of 1.88 Å (H···O) and 2.68 Å (O–H···O) on average to guest molecules [41-44]. BIFOXSi(OH)2 (9, Figure 16) binds an acetone in a similar manner (O4’–H···O4–H···O6=C(CH3)2) with distances of 1.88(3) Å (H4···O6) and 2.62(1) Å (O4–H···O6) as well as 2.05(2) Å (H4···O4’) and 2.73(1) Å (O4–H···O4’) between the hydroxy groups. The second acetone is bonded with one hydroxy group of each of the BIFOXSi(OH)2 (9) with longer distances of 2.03(0) Å (H···O) and 2.80(3) Å (O–H···O) (Table 5). Similarly to the previously reported silanediol derivatives 2a and 2b [43], an increase of acidity of one outward facing OH group is achieved by intermolecular hydrogen bonds [43].
Table 5: Bond lengths and angles of hydrogen bonds in X-ray crystal structures of BIFOXSiCl(OH) (8) and BIFOXSi(OH)2 (9).
D–H···A | D (D···A)a [Å] | D (H···A)b [Å] |
|
|
---|---|---|---|---|
BIFOXSi(OH)2 (9)
(Figure 14) |
O4H–O3 | 2.76(1) | 1.92(0) | 170.0(5) |
BIFOXSi(OH)2 (9)
(Figure 15) |
O4H–O3’
O4’H–O4’’ O4’’H–O4 O3H–O3’’ O3’’H–O4’’’ O4’’’H–O3 |
2.76(2)
2.73(5) 2.66(4) 2.68(3) 2.79(9) 2.74(7) |
1.98(0)
1.95(0) 1.82(4) 1.85(8) 2.02(1) 2.02(4) |
163.7(4)
172.0(7) 165.5(0) 168.9(8) 152.4(6) 165.9(3) |
BIFOXSi(OH)2 (9)
(Figure 16) |
O3H–O5
O4H–O4’ O4H–O6 |
2.80(3)
2.73(1) 2.62(1) |
2.03(0)
2.05(2) 1.88(3) |
161.1(8)
176.2(8) 146.3(6) |
BIFOXSiCl(OH) (8)
(Figure 17) |
O3H–O4 | 2.89(4) | 2.16(0) | 167.1(8) |
aDistance for hydrogen bond donor (D) to hydrogen bond acceptor (A). bDistance for hydrogen (H) to hydrogen bond acceptor (A).
Chloride binding
The X-ray crystal structures of chlorosilanol 8 (Figure 17) and silanediol 9 (Figure 16) with co-crystallized acetone indicate the ability of binding ions or molecules via hydrogen bonds. To investigate this ability, UV–vis titration experiments with tetrabutylammonium chloride (TBA-Cl) are carried out, with silanediol 1 as a reference (Table 6) [47,75]. For BIFOXSi(OH)2 (9, Figure 14, Figure 15) a binding constant of 5274.9 M−1 (13%) for chloride is determined, which is in the same order as di(1-naphthyl)silanediol (1, Figure 1) with 4688.0 M−1 (5%, Table 6). The binding constant of chlorosilanol 8 (Figure 13) for chloride is 451.1 M−1 (4%). Thus chlorosilanol 8 and silanediol 9 are feasible for anion binding of chloride.
Table 6: Chloride binding constants (K [M−1], error (%)) to BIFOXSiCl(OH) (8), BIFOXSi(OH)2 (9) and di(1-naphthyl)silanediol (1) in HCCl3 at 22 °C [75].
8 | 9 | 1 | |
binding constanta | 451.1 (4%) | 5274.9 (13%) | 4688.0 (5%) |
aCalculated for 1:1 binding mode between chloride and hydrogen bond donor [75].
Counter ion catalyses
The N-acyl Mannich reaction of isochinolin (16), which is activated with 2,2,2-trichloroethoxycarbonyl chloride (17, TrocCl) to carbamate 10, and different silyl ketene acetals 11a–d yielding product 12 (Scheme 6) [45,47], is studied. Mattson et al. proposed a mechanism where the chloride ion is abstracted from 10 and binds via hydrogen bonding to the catalyst (Scheme 6) [45,47]. This leads to an ion pair [cat•Cl]− and [isoquinolinium cation]+ (Scheme 6). The nucleophilic silyl ketene acetal reacts with the [isoquinolinium cation]+ and forms the C–C bond, yielding product 12 (Scheme 6).
Scheme 6: Hydrogen-bond-catalyzed N-acyl Mannich reaction of in situ-generated 10 with different silyl ketene acetals 11 a–d and BIFOXSi(OH)2 (9) yielding C–C coupling product 12 (Tables 6–8); (TMS: trimethylsilane. TES: triethylsilane. TIPS: triisopropylsilane. TBDMS: tert-butyldimethylsilane).
Scheme 6: Hydrogen-bond-catalyzed N-acyl Mannich reaction of in situ-generated 10 with different silyl ketene...
The reactivity and stability of such an ion pair depend on the employed solvent. For this reaction and BIFOXSi(OH)2 (9) as catalyst, several solvents are tested (Table 7). In a nonpolar solvent like n-hexane, no catalytic activity is observed (Table 7, entry 1). In halogenated solvents as dichloromethane (DCM) and 1,2-dichloroethane (1,2-DCE) the reaction takes place but without any enantiomeric excess (ee) (Table 7, entries 2 and 3). In DCM the highest yield is isolated, but that is due to a fast background reaction [45]. With toluene as solvent, no background reaction is observed (Table 9, entry 9). To stabilize and improve the ion pair, polar solvents are tested as diethyl ether and dimethylformamide gave no conversion and starting material is obtained (Table 7, entries 4 and 5), acetonitrile and acetone increase the yield (Table 7, entries 6 and 7), but without any enantiomeric excess. In toluene, BIFOXSi(OH)2 (9), forms 43% yield at −80 °C and 5% ee (Table 7, entry 8). At higher temperature, 12 is isolated with 52% yield and 12% ee (−60 °C, Table 7, entry 9). Further aromatic solvents are tested (Table 7, entries 10–13), but without any improvement in yield or ee.
Table 7: N-acyl Mannich reaction of 10 and 11c catalyzed by silanediol 9 yielding product 12 with different solvents (Scheme 6)a.
entry | solvent | T [°C] | yield [%]b | ee [%]c |
---|---|---|---|---|
1 | n-hexane | −60 | 0 | – |
2 | DCM | −60 | 87 | 0 |
3 | 1.2-DCE | −30 | 33 | 0 |
4 | diethyl ether | −60 | 0 | – |
5 | dimethylformamide | −40 | 0 | – |
6 | acetonitrile | −40 | 35 | 1 |
7 | acetone | −80 | 10 | 0 |
8 | toluene | −80 | 43 | 5 |
9 | toluene | –60 | 52 | 12 |
10 | benzene | rt | 10 | 1 |
11 | m-xylene | −60 | <1 | 12 |
12 | nitrobenzene | rt | 40 | 0 |
13 | pyridine | −30 | 0 | – |
aReactions carried out with 20 mol % 9, 0.1 mmol 16, 0.11 mmol 17 and 0.15 mmol 11c in 4 mL solvent; bisolated yields; cchiral HPLC OD-H, n-hexane/iPrOH 90:10, 1 mL/min, 220 nm, 25 °C, (−)-12 correlates to (S)-12 [45,76].
Variation of the catalyst loading suggests a ratio of 10 mol % of BIFOXSi(OH)2 (9, Table 8, entries 1–4) to be optimal. An increase of temperature results in decreasing yields and ee (Table 8, entries 5–8). The highest ee is found with 20 mol % catalyst (12% ee, 52% yield, Table 7, entry 9).
Table 8: Different catalyst loadings of BIFOXSi(OH)2 (9) and different temperatures in the N-acyl Mannich reaction of 10 and 11c yielding product 12 (Scheme 6)a.
entry | cat loading [mol %] | T [°C] | yield [%]b | ee [%]c |
---|---|---|---|---|
1 | 20 | −80 | 43 | 5 |
2 | 10 | −80 | 52 | 7 |
3 | 5 | −80 | 12 | 0 |
4 | 1 | −80 | 2 | 2 |
5 | 10 | −60 | 37 | 5 |
6 | 10 | −40 | 18 | 7 |
7 | 10 | 0 | 9 | 1 |
8 | 10 | rt | 20 | 0 |
aReactions carried out with 0.1 mmol 16, 0.11 mmol 17 and 0.15 mmol 11c in 4 mL toluene; bisolated yields, cchiral HPLC OD-H, n-hexane/iPrOH 90:10, 1 mL/min, 220 nm, 25 °C, (−)-12 correlates to (S)-12 [45,76].
BIFOXSi(OH)2 (9) performs better than BIFOXSiCl(OH) (8) which is in accordance with the determined binding constant for chloride (Table 6, Table 9). With BIFOXSiCl(OH) (8) a yield up to 60% is isolated (Table 9, entry 3), but as racemate. BIFOXSi(OH)2 (9) catalyses the reaction with good yields up to 73% and an ee value of 12% (Table 9, entry 7). For 11a, b and d and silanediol 9 as catalyst, an enantiomeric inversion is observed (Table 9, entries 5, 6 and 8).
Table 9: Performance of catalyst 8 and 9 in the N-acyl Mannich reaction with 10 and different silyl ketene acetals 11a–d and yielding in 12 (Scheme 6)a.
entry | catalyst | SiR3 | yield [%]b | ee [%]c |
---|---|---|---|---|
1 | 8 | 11a TMS | 56 | 3 S-12 |
2 | 8 | 11b TES | 42 | 4 S-12 |
3 | 8 | 11c TIPS | 60 | 4 S-12 |
4 | 8 | 11d TBDMS | 46 | 2 S-12 |
5 | 9 | 11a TMS | 73 | 6 R-12 |
6 | 9 | 11b TES | 67 | 2 R-12 |
7 | 9 | 11c TIPS | 52 | 12 S-12 |
8 | 9 | 11d TBDMS | 72 | 2 R-12 |
9 | – | 11c TIPS | 0 | – |
aReactions carried out with 0.1 mmol 16, 0.11 mmol 17, 0.15 mmol 11a–d and 20 mol % cat in 4 mL toluene at −60 °C; bisolated yields; cchiral HPLC OD-H, n-hexane/iPrOH 90:10, 1 mL/min, 220 nm, 25 °C, (−)-12 correlates to (S)-12 [45,76].
The substrate scope is broadened with 1-chloroisochroman (18) as alternative substrate (Table 10). The reaction mechanism is analogue to the N-acyl Mannich reaction (Scheme 6 vs Scheme 7). The catalyst abstracts and binds the chloride anion and forms an ion pair [cat•Cl]− and oxocarbenium ion [18]+. Silyl ketene acetal 11 reacts with this ion pair complex to product 19 [77,78]. Only with DCM as solvent, product 19 of the reaction has been isolated (Table 10). Silanediol 9 and silyl ketene acetal 11a provide the highest yield (85%, Table 10, entry 5). The substitution pattern on the silyl ketene has a direct influence on the yield.
Scheme 7: Hydrogen-bond-catalyzed nucleophilic substitution of 18 with BIFOXSi(OH)2 (9) and nucleophile silyl ketene acetals 11. 18 and 9 form an activated electrophile ion pair complex which yields C–C coupling product 19 (Table 10).
Scheme 7: Hydrogen-bond-catalyzed nucleophilic substitution of 18 with BIFOXSi(OH)2 (9) and nucleophile silyl...
The highest yield is reached with TMS substitution (silanediol 9, 85% yield, Table 10, entry 5; chlorosilanol 8, 54% yield, Table 10, entry 9). The yield decreases as the substituents become larger (Table 10, entries 6–8, 10 and 11). This trend can also be seen in the uncatalyzed reaction (Table 10, entries 13, 14). Only for 11c and silandiol 9 a considerable ee with 5% is determined (Table 10, entries 7 and 11). Chlorosilanol 8 does not show catalytic activity for the reaction of 18 with 11a, as the background reaction is slightly faster (54% vs 58%, Table 10, entries 9 and 13). With increasing of the steric demand of the nucleophilic silyl group, the background reaction slows down and chlorosilanol 8 has a positive influence on the yields and the enantiomeric excess.
Table 10: Hydrogen-bond-catalyzed addition of silyl ketene acetals 11a–d with 1-chloroisochroman (18) to product 19 with chlorosilanol 8 and silanediol 9 in different solventsa (Scheme 7).
entry | cat. | solvent | SiR3 | yield [%]b | ee [%]c |
---|---|---|---|---|---|
1 | 9 | toluene | 11a TMS | 0 | – |
2 | 9 | MTBE | 11a TMS | 0 | – |
3 | 9 | THF | 11a TMS | 0 | – |
4 | 9 | diethyl ether | 11a TMS | 0 | – |
5 | 9 | DCM | 11a TMS | 85 | 1 |
6 | 9 | DCM | 11b TES | 37 | 3 |
7 | 9 | DCM | 11c TIPS | 12 | 5 |
8 | 9 | DCM | 11d TBDMS | 8 | 3 |
9 | 8 | DCM | 11a TMS | 54 | 0 |
10 | 8 | DCM | 11b TES | 46 | 2 |
11 | 8 | DCM | 11c TIPS | 16 | 5 |
12 | 8 | DCM | 11d TBDMS | 12 | 3 |
13 | – | DCM | 11a TMS | 58 | – |
14 | – | DCM | 11c TIPS | 5 | – |
aReactions carried out with 0.15 mmol 18, 0.22 mmol 11a–d and 20 mol % cat in 1.2 mL solvent at −60 °C; bisolated yields; cchiral HPLC OD-H, n-hexane/iPrOH 100:0, 1.0 mL/min, 210 nm, 25 °C, (−)-18 correlates to (S)-18 [77].
In a third reaction, the 1,4 addition of silyl keten acetals 11 to chromone 20 is investigated (Table 11, Scheme 8). Chromone 20 is first transformed to the oxonium ion pair 21. Catalyst BIFOXSi(OH)2 (9) binds the triflate anion via hydrogen bonding and leaves the pyrylium derivative 21 for the nucleophilic attack of silyl keten acetals 11 to form product 22 (Scheme 8).
Scheme 8: Nucleophilic substitution of 20 with BIFOXSi(OH)2 (9) and nucleophile silyl ketene acetals 11, 20 and 9 form an activated electrophile ion pair complex which yields C–C coupling product 22 (Table 11).
Scheme 8: Nucleophilic substitution of 20 with BIFOXSi(OH)2 (9) and nucleophile silyl ketene acetals 11, 20 a...
With BIFOXSi(OH)2 (9) an increase of yield (71% yield, 4% ee, Table 11, entry 2) compared to the not catalyzed reaction (48%, Table 11, entry 10) is achieved. BIFOXSiCl(OH) (8) has no activation ability, but little effect on the ee (46% yield, 1% ee, Table 11, entry 6). Here, the sterically demanding silyl keten acetals increase yields. With BIFOXSi(OH)2 (9), the bulkiest acetal 11d yields 98% and the smallest 11a yields 60% of product 22 (Table 11, entries 1–4). This tendency is the same for BIFOXSiCl(OH) (8, Table 11, entries 5–8), with exception of acetal 11a, which yields 55% of product 22. Mattson et al. used 2,6-di-tert-butyl-4-methylpyridine as additive [48]. It is added at the beginning to the reaction, so it should support the formation of the ion pair 21. As organic base it binds also to BIFOXSi(OH)2 (9), which results in a lower yield and ee (Table 11, entry 9).
Table 11: Hydrogen-bond-catalyzed addition of silyl ketene acetals 11a–d with chromone 20 to product 22 with chlorosilanol 8 and silanediol 9 in toluenea (Scheme 8).
entry | catalyst | SiR3 | yield [%]b | ee [%]c |
---|---|---|---|---|
1 | 9 | 11d TBDMS | 98 | 1 |
2 | 9 | 11c TIPS | 71 | 4 |
3 | 9 | 11b TES | 69 | 1 |
4 | 9 | 11a TMS | 60 | 0 |
5 | 8 | 11d TBDMS | 70 | 0 |
6 | 8 | 11c TIPS | 46 | 1 |
7 | 8 | 11b TES | 35 | 1 |
8 | 8 | 11a TMS | 55 | 1 |
9d | 9 | 11c TIPS | 50 | 2 |
10e | – | 11c TIPS | 48 | – |
aReactions carried out with 0.15 mmol 20, 0.22 mmol 11a–d and 20 mol % cat in 4 mL toluene at −80°C; bisolated yields; cchiral HPLC AD-H, n-hexane/iPrOH 98:2, 1 mL/min, 254 nm, 25 °C, (+)-22 correlates to (S)-22 [48]; dwith 20 mol % 2.6-di-tert-butyl-4-methylpyridine as additive for step 1 (Scheme 8); ewithout catalyst.
Conclusion
Enantiopure fenchole-based silanediol BIFOXSi(OH)2 (9, Figure 1) and chlorosilanol BIFOXSiCl(OH) (8, Figure 1) are efficiently accessible from BIFOL (5) via enforced hydrolysis of dichlorosilane 7, i.e., H2O/THF reflux, 19 h (Scheme 3, Figure 2).
DFT computations reveal two different hydrolysis mechanisms and explain the unusual low reactivity of BIFOXSiCl2 (7) and BIFOXSiCl(OH) (8, Table 3) with sterically demanding endo fenchone groups. For BIFOXSiCl(OH) (8) two isomers (8eq vs 8ax) are found computationally. Chlorosilanol 8ax, with the axial Si–OH alignment, is the thermodynamically more stable isomer (ΔEr = 2.7 kcal mol−1, Table 3), in accordance with X-ray crystal structure analyses of 8 (Figure 13). The first hydrolysis has a higher activation barrier than the second step, and thus appears to be rate-determining.
In the X-ray crystal structures of BIFOXSiCl(OH) (8, Figure 13) and BIFOXSi(OH)2 (9, Figures 14–16), intermolecular hydrogen bonds are apparent. The lengths of these hydrogen bonds vary from 1.88(3) to 2.16(2) Å (Table 5). The longest bond appears between BIFOXSiCl(OH) (8) and acetone (Figure 17). This suggests that BIFOXSiCl(OH) (8) is the weaker hydrogen bond donor, compared to BIFOXSi(OH)2 (9). This is additionally supported by UV–vis titrations of chloride with BIFOXSiCl(OH) (8, 451.1 (4%) M−1) and BIFOXSi(OH)2 (9, 5274.9 (13%) M−1, Table 6).
Both new hydrogen bond catalysts can be used for the C–C coupling in the N-acyl Mannich reaction with activated isochinolin 10, 1-chloroisochroman (18) and chromone 21 with different silyl ketene acetals. Due to more efficient bifunctional Si(OH)2-hydrogen bonding, silandiol 9 tops chlorosilanol 8, also on catalytic application.
Computational Details
In this work computations were performed using GAUSSIAN 09 [79]. Geometry optimizations and frequency computations were performed at the B3LYP-D3BJ/6-31G(d) level of theory. Zero-point energies were scaled by 0.96 [80]. Single point energies were performed at the M06-2X-D3/6-311++G(d,p) level of theory using the PCM method.
Experimental
General considerations: All reactions were carried out under an argon atmosphere by using Schlenk techniques, unless otherwise stated. Solvents used in chemical conversions were dried by standard methods and distilled under argon prior to use unless otherwise specified. NMR spectra were recorded on a Bruker Avance II 300 instrument. UV–vis spectra were recorded by using a Perkin Elmer Lambda35 spectrometer. The samples were placed in quartz cells of 1 cm path length. NMR spectra, UV–vis spectra, crystal data and the coordinates of computed stationary points/transition states, as well as experimental details can be found in Supporting Information File 1. CCDC-1833170 (BIFOXSiCl2 (7)), CCDC-1833171 (BIFOXSiCl(OH) (8)), CCDC-1833172 (BIFOXSi(OH)2 (9, dimer)), CCDC-1833173 (BIFOXSi(OH)2 (9, tetramer)), CCDC-1833174 (BIFOXSiCl(OH) 8·acetone) and CCDC-1833175 (BIFOXSi(OH)2 9·acetone) contain the supplementary crystallographic data for this paper. These data can be obtained free of charge from The Cambridge Crystallographic Data Centre via http://www.ccdc.cam.ac.uk/data_request/cif.
Synthesis of BIFOXSiCl2 (7): In a dried Schlenk flask BIFOL (5, 2.3 g, 5 mmol. 1 equiv) was solved in THF (25 mL) in an inert gas atmosphere. After cooling to 0 °C, n-BuLi (4 mL, 10 mmol, 2 equiv, 2.5 M in n-hexane) was slowly added. The solution was stirred for 30 min und was allowed to warm up to 20 °C. After cooling to 0 °C again tetrachlorosilane (2.86 mL, 25 mmol, 5 equiv) was added dropwise. The reaction mixture was warmed to 20 °C and stirred overnight. Aqueous work-up with saturated NH4Cl followed by extracting with Et2O (three times) and concentrated in vacuo results in crude product. After purification with silica gel flash column chromatography (100% n-hexane, Rf: 0.58) BIFOXSiCl2 (7) was obtained as a white solid (2.5 g, 4.6 mmol, 92%); mp 219.4 °C; [α]43620 = −54.90 (c 0.46, CHCl3); 1H NMR (300 MHz, CDCl3, 25 °C, TMS) δ 7.61 (dd, J = 8.1, 1.1 Hz, 2H), 7.26–7.19 (m, 2H), 7.14–7.06 (m, 2H), 6.97 (dd, J = 7.7, 1.6 Hz, 2H), 2.46–2.32 (m, 4H), 1.72–1.60 (m, 4H), 1.56 (s, 6H), 1.46–1.35 (m, 4H), 1.25 (td, J = 5.2 Hz, 2H), 0.64 (s, 6H), 0.49 (s, 6H); 13C NMR (75 MHz, CDCl3, 25 °C, TMS) δ 143.20, 141.37, 136.14, 128.83, 125.06, 124.63, 94.59, 55.33, 50.19, 48.39, 44.06, 35.91, 28.89, 23.47, 20.80, 20.74; 29Si NMR (60 MHz, CDCl3, 25 °C, TMS) δ −21.92.
Synthesis of BIFOXSiCl(OH) (8): In a dried Schlenk flask BIFOXSiCl2 (7, 1 g, 1.8 mmol, 1 equiv) was solved in THF (10 mL). Then triethylamine (0.52 mL, 3.6 mmol, 2 equiv) and H2O (0.032 mL, 1.8 mmol, 1 equiv) was added. The reaction mixture was stirred for 20 h at 20 °C and concentrated in vacuo. The residue was purified by silica gel flash column chromatography (n-hexane/ethyl acetate: 9:0.5, Rf: 0.43) which resulted in BIFOXSiCl(OH) (8, 642.6 mg, 1.2 mmol, 67%) as a white solid and BIFOXSi(OH)2 (9, 90.6 mg. 0.1 mmol. 6%) as a white solid; mp 105.7–112.5 °C; [α]58920 = −3.24 (c 0.658, CHCl3); 1H NMR (300 MHz, CDCl3, 25 °C, TMS) δ 7.73 (d, J = 8.0 Hz, 1H), 7.61 (dd, J = 7.6, 1.7 Hz, 1H), 7.35–7.17 (m, 4H), 7.03 (t, J = 7.3 Hz, 1H), 6.72 (dd, J = 7.8, 1.5 Hz, 1H), 2.66–2.48 (m, 2H), 2.40 (s, 1H), 2.31 (d, J = 10.5 Hz, 1H), 1.72 (s, 3H), 1.61 (s, 5H), 1.52 (s, 3H), 1.51–1.20 (m, 6H), 0.90 (s, 3H), 0.82 (s, 3H), 0.45 (s, 3H), 0.08 (s, 3H); 13C NMR (75 MHz, CDCl3, 25 °C, TMS) δ 145.10, 144.39, 142.08, 140.14, 137.33, 133.86, 129.25, 129.05, 128.23, 125.63, 125.25, 124.60, 123.70, 92.83, 92.25, 56.38, 54.22, 50.96, 49.57, 49.31, 46.78, 43.87, 43.78, 35.80, 35.02, 29.45, 28.36, 23.86, 23.42, 22.28, 21.49, 20.63, 19.33, 19.18; 29Si NMR (60 MHz, CDCl3, 25 °C, TMS) δ −21.93; MS (HRMS ESI) m/z: [M + Na]+ calcd for C32H41O3ClNaSi, 559.2405; found, 559.2404 (−0.1 ppm).
Synthesis BIFOXSi(OH)2 (9): In a dried Schlenk flask BIFOXSiCl2 (7, 1 g, 1.8 mmol, 1 equiv) was solved in THF (25 mL) and H2O (25 mL). The solution was heated to reflux and stirred overnight. Then the solution was concentrated in vacuo and purified by silica gel flash column chromatography (n-hexane/ethyl acetate 9:1, Rf: 0.26). BIFOXSi(OH)2 (9) was obtained as white solid (0.78 g, 1.5 mmol, 84%); mp 199.4 °C; [α]58920 = 24.02 (c 0.769, CHCl3); 1H NMR (300 MHz, CDCl3, 25 °C, TMS) δ 7.60 (d, J = 8.0 Hz, 2H), 7.20 (td, J = 7.8, 1.7 Hz, 2H), 7.07 (td, J = 7.5, 1.1 Hz, 2H), 6.95 (dd, J = 7.7, 1.5 Hz, 2H). 2.48–2.19 (m, 4H), 2.03 (d, J = 2.5 Hz, 2H), 1.70–1.57 (m, 4H), 1.49 (s, 6H), 1.43–1.30 (m, 4H), 1.18 (td, J = 12.5, 4.8 Hz, 2H), 0.59 (s, 6H), 0.45 (s, 6H); 13C NMR (75 MHz, CDCl3, 25 °C, TMS) δ 144.28, 141.97, 135.16, 128.80, 124.70, 124.17, 90.24, 55.04, 50.08, 48.11, 43.98, 35.33, 29.01, 23.65, 20.85, 19.76; MS (HRMS ESI) m/z: [M + Na]+ calcd for C32H42O4NaSi, 541.2744; found, 541.2742 (−0.4 ppm); 29Si NMR (60 MHz, CDCl3, 25 °C, TMS) δ −21.92.
General procedure for the hydrolysis studies of dichlorosilanes 7, 13 and 14: Dichlorosilane (0.09 mmol) was solved in THF (2.5 mL) or THF/H2O (1.25 mL/1.25 mL). For THF/H2O/KOH conditions, KOH (0.9 mmol, 50.5 mg, 10 equiv) was added. The reaction mixture was heated and stirred as stated. After the reaction time the mixture was extracted two times with diethyl ether (2 mL) and concentrated in vacuo. The residue was solved in THF (5 mL). A sample (0.5 mL) was transferred to a GC vial and n-tetradecane solution (0.01 M in THF, 0.5 mL) was added as standard for GC analysis.
General procedure for the N-acyl Mannich reaction of isoquinolin 16 with silyl ketene acetals 11 to product 12: In a heat dried Schlenk tube isoquinolin (16, 11 μL, 0.1 mmol, 1 equiv) was solved in solvent (4 mL) and cooled to 0 °C under inert gas atmosphere. To this solution 2,2,2-trichlorethoxycarbonyl chloride (15 μL, 0.11 mmol, 1.1 equiv) was added. The cooling was removed. The solution warmed to 20 °C and stirred for 30 min. After this the solution was cooled to reaction temperature. The catalyst was added and stirred for 10 minutes. Then silyl ketene acetall 11 (0.15 mmol, 1.5 equiv) was added and the reaction mixture stirred for 6 h. The reaction was quenched by adding NaOMe (0.2 mL, 0.5 M in MeOH), filtered through silica gel with ethyl acetate as eluent and concentrated in vacuo. After further purification by silica gel flash column chromatography (n-hexane/ethyl acetate 95:5) product 12 was obtained. The enantiomeric excess is determined by chiral HPLC analysis (see Supporting Information File 1).
General procedure for addition of silyl ketene acetals 11 to 1-chloroisochroman (18) to product 19: In a heat dried Schlenk tube 1-chloroisochroman (18, 0.15 mmol, 0.3 mL of 0.5 M in toluene) was solved in solvent (1.2 mL) under inert gas atmosphere and cooled to −60 °C. After this catalyst (0.03 mmol, 0.2 equiv) was added and stirred for 10 min. Then silyl ketene acetal 11 (0.22 mmol, 1.5 equiv) was added and the resulting reaction mixture was stirred for 6 h. The reaction was quenched by adding NaOMe (0.2 mL, 0.5 M in MeOH), concentrated in vacuo and purified by silica gel flash column chromatography (n-hexane/Et2O 9:1). The enantiomeric excess is determined by chiral HPLC analysis (see Supporting Information File 1).
General procedure for addition of silyl ketene acetals 11 to chromone 20 to product 22: In a heat dried Schlenk tube chromone 20 (14.6 mg, 0.1 mmol, 1 equiv) was solved in 2 mL dried toluene under inert gas atmosphere. TIPSOTf (29.5 μL, 0.11 mmol, 1.1 equiv) was added and heated to 60 °C for 1 h. After this, the reaction mixture was cooled to −80 °C, catalyst (0.02 mmol, 0.2 equiv) and silyl ketene acetal 11 (0.14 mmol, 1.25 equiv), solved in 2 mL dried toluene, were added. The resulting reaction mixture was stirred for 4 h. The reaction was quenched by adding 3 M HCl (0.2 mL), concentrated in vacuo and purified by silica gel flash column chromatography (n-hexane/ethyl acetate 9:1). The enantiomeric excess is determined by chiral HPLC analysis (see Supporting Information File 1).
Supporting Information
Supporting Information File 1: Copies of all NMR spectra, HPLC graphs, GC graphs of the kinetic study. | ||
Format: PDF | Size: 2.4 MB | Download |
Acknowledgements
We thank the Fonds der Chemischen Industrie, the Deutsche Forschungsgemeinschaft (DFG GO-930-13), Bayer AG, BASF AG, Wacker AG, Evonic AG, Raschig GmbH, Symrise GmbH, Solvay GmbH, the OMG group, and INEOS-Köln for support. We also thank the computing center of the University of Cologne (RRZK) for providing CPU time on the DFG-funded supercomputer CHEOPS, as well as for support. Special thanks go to Niklas Kolks and Matthias Spilles for their contributions during their practical courses.
References
-
Chandrasekhar, V.; Boomishankar, R.; Nagendran, S. Chem. Rev. 2004, 104, 5847–5910. doi:10.1021/cr0306135
Return to citation in text: [1] [2] -
Hernández, D.; Mose, R.; Skrydstrup, T. Org. Lett. 2011, 13, 732–735. doi:10.1021/ol102968g
Return to citation in text: [1] [2] -
Kim, J. K.; Sieburth, S. M. J. Org. Chem. 2012, 77, 2901–2906. doi:10.1021/jo300175t
Return to citation in text: [1] -
Madsen, J. L. H.; Andersen, T. L.; Santamaria, S.; Nagase, H.; Enghild, J. J.; Skrydstrup, T. J. Med. Chem. 2012, 55, 7900–7908. doi:10.1021/jm301000k
Return to citation in text: [1] [2] -
Guan, Y.; Attard, J. W.; Visco, M. D.; Fisher, T. J.; Mattson, A. E. Chem. – Eur. J. 2018, 24, 7123–7127. doi:10.1002/chem.201801304
Return to citation in text: [1] -
Visco, M. D.; Attard, J.; Guan, Y.; Mattson, A. E. Tetrahedron Lett. 2017, 58, 2623–2628. doi:10.1016/j.tetlet.2017.05.045
Return to citation in text: [1] [2] -
Plunkett, M. J.; Ellman, J. A. J. Org. Chem. 1995, 60, 6006–6007. doi:10.1021/jo00124a005
Return to citation in text: [1] -
Anderson, T. F.; Statham, M. A. J.; Carroll, M. A. Tetrahedron Lett. 2006, 47, 3353–3355. doi:10.1016/j.tetlet.2006.03.095
Return to citation in text: [1] -
Glekas, A.; Sieburth, S. M. Tetrahedron Lett. 2001, 42, 3799–3801. doi:10.1016/s0040-4039(01)00576-7
Return to citation in text: [1] -
Duong, H. Q.; Sieburth, S. M. J. Org. Chem. 2018, 83, 5398–5409. doi:10.1021/acs.joc.8b00116
Return to citation in text: [1] -
Yao, C.; Yu, J.; Wang, Y.; Tang, C.; Huang, C. Dent. Mater. 2018, 34, 809–818. doi:10.1016/j.dental.2018.02.004
Return to citation in text: [1] -
Carilla, J.; Fajarí, L.; Juliá, L.; Riera, J.; Lloveras, J.; Verdaguer, N.; Fita, I. J. Organomet. Chem. 1992, 423, 163–171. doi:10.1016/0022-328x(92)83111-t
Return to citation in text: [1] -
Bruña, S.; Garrido-Castro, A. F.; Perles, J.; Montero-Campillo, M. M.; Mó, O.; Kaifer, A. E.; Cuadrado, I. Organometallics 2016, 35, 3507–3519. doi:10.1021/acs.organomet.6b00559
Return to citation in text: [1] -
Auner, N.; Probst, R.; Heikenwälder, C.-R.; Herdtweck, E.; Gamper, S.; Müller, G. Z. Naturforsch., B: J. Chem. Sci. 1993, 48, 1625–1634. doi:10.1515/znb-1993-1123
Return to citation in text: [1] -
Belzner, J.; Schär, D.; Herbst-Irmer, R.; Kneisel, B. O.; Noltemeyer, M. Tetrahedron 1998, 54, 8481–8500. doi:10.1016/s0040-4020(98)00451-7
Return to citation in text: [1] -
Hurkes, N.; Belaj, F.; Koe, J. R.; Pietschnig, R. Appl. Organomet. Chem. 2018, 32, e4427. doi:10.1002/aoc.4427
Return to citation in text: [1] -
Shamaev, A. E.; Glazkov, A. A.; Ignatenko, A. V.; Sakharov, A. M. Russ. Chem. Bull. 2005, 54, 1250–1253. doi:10.1007/s11172-005-0389-y
Return to citation in text: [1] -
Safa, K. D.; Asadi, A.; Sargordan, M. J. Organomet. Chem. 1997, 545–546, 61–65. doi:10.1016/s0022-328x(97)00302-1
Return to citation in text: [1] -
George, P. D.; Sommer, L. H.; Whitmore, F. C. J. Am. Chem. Soc. 1953, 75, 1585–1588. doi:10.1021/ja01103a020
Return to citation in text: [1] -
Cusa, N. W.; Kipping, F. S. J. Chem. Soc. 1932, 2205–2209. doi:10.1039/jr9320002205
Return to citation in text: [1] -
Ojima, I.; Fuchikami, T.; Yatabe, M. J. Organomet. Chem. 1984, 260, 335–346. doi:10.1016/s0022-328x(00)99483-x
Return to citation in text: [1] -
Robinson, R.; Kipping, F. S. J. Chem. Soc., Trans. 1912, 101, 2142–2155. doi:10.1039/ct9120102142
Return to citation in text: [1] -
Shaffer, L. H.; Flanigen, E. M. J. Phys. Chem. 1957, 61, 1591–1595. doi:10.1021/j150558a004
Return to citation in text: [1] -
Shaffer, L. H.; Flanigen, E. M. J. Phys. Chem. 1957, 61, 1595–1600. doi:10.1021/j150558a005
Return to citation in text: [1] -
Swain, C. G.; Esteve, R. M.; Jones, R. H. J. Am. Chem. Soc. 1949, 71, 965–971. doi:10.1021/ja01171a056
Return to citation in text: [1] -
Doyle, A. G.; Jacobsen, E. N. Chem. Rev. 2007, 107, 5713–5743. doi:10.1021/cr068373r
Return to citation in text: [1] -
Taylor, M. S.; Jacobsen, E. N. Angew. Chem., Int. Ed. 2006, 45, 1520–1543. doi:10.1002/anie.200503132
Return to citation in text: [1] [2] -
Auvil, T. J.; Schafer, A. G.; Mattson, A. E. Eur. J. Org. Chem. 2014, 2633–2646. doi:10.1002/ejoc.201400035
Return to citation in text: [1] [2] [3] -
Alemán, J.; Parra, A.; Jiang, H.; Jørgensen, K. A. Chem. – Eur. J. 2011, 17, 6890–6899. doi:10.1002/chem.201003694
Return to citation in text: [1] -
Zhang, Z.; Schreiner, P. R. Chem. Soc. Rev. 2009, 38, 1187–1198. doi:10.1039/b801793j
Return to citation in text: [1] -
Takemoto, Y. Chem. Pharm. Bull. 2010, 58, 593–601. doi:10.1248/cpb.58.593
Return to citation in text: [1] -
Klare, H.; Neudörfl, J. M.; Goldfuss, B. Beilstein J. Org. Chem. 2014, 10, 224–236. doi:10.3762/bjoc.10.18
Return to citation in text: [1] -
Klare, H.; Hanft, S.; Neudörfl, J. M.; Schlörer, N. E.; Griesbeck, A.; Goldfuss, B. Chem. – Eur. J. 2014, 20, 11847–11855. doi:10.1002/chem.201403013
Return to citation in text: [1] -
Wolf, F. F.; Klare, H.; Goldfuss, B. J. Org. Chem. 2016, 81, 1762–1768. doi:10.1021/acs.joc.5b02167
Return to citation in text: [1] -
Wolf, F. F.; Neudörfl, J.-M.; Goldfuss, B. New J. Chem. 2018, 42, 4854–4870. doi:10.1039/c7nj04660j
Return to citation in text: [1] -
Kondo, S.-i.; Okada, N.; Tanaka, R.; Yamamura, M.; Unno, M. Tetrahedron Lett. 2009, 50, 2754–2757. doi:10.1016/j.tetlet.2009.03.134
Return to citation in text: [1] -
Busschaert, N.; Caltagirone, C.; Van Rossom, W.; Gale, P. A. Chem. Rev. 2015, 115, 8038–8155. doi:10.1021/acs.chemrev.5b00099
Return to citation in text: [1] -
Brak, K.; Jacobsen, E. N. Angew. Chem., Int. Ed. 2013, 52, 534–561. doi:10.1002/anie.201205449
Return to citation in text: [1] -
Kondo, S.-i.; Harada, T.; Tanaka, R.; Unno, M. Org. Lett. 2006, 8, 4621–4624. doi:10.1021/ol061822p
Return to citation in text: [1] [2] [3] -
Liu, M.; Tran, N. T.; Franz, A. K.; Lee, J. K. J. Org. Chem. 2011, 76, 7186–7194. doi:10.1021/jo201214x
Return to citation in text: [1] [2] -
Tran, N. T.; Min, T.; Franz, A. K. Chem. – Eur. J. 2011, 17, 9897–9900. doi:10.1002/chem.201101492
Return to citation in text: [1] [2] [3] [4] [5] -
Tran, N. T.; Wilson, S. O.; Franz, A. K. Org. Lett. 2012, 14, 186–189. doi:10.1021/ol202971m
Return to citation in text: [1] [2] [3] [4] [5] -
Tran, N. T.; Wilson, S. O.; Franz, A. K. Chem. Commun. 2014, 50, 3738–3740. doi:10.1039/c4cc00672k
Return to citation in text: [1] [2] [3] [4] [5] [6] [7] -
Wilson, S. O.; Tran, N. T.; Franz, A. K. Organometallics 2012, 31, 6715–6718. doi:10.1021/om300736n
Return to citation in text: [1] [2] [3] [4] -
Schafer, A. G.; Wieting, J. M.; Fisher, T. J.; Mattson, A. E. Angew. Chem., Int. Ed. 2013, 52, 11321–11324. doi:10.1002/anie.201305496
Return to citation in text: [1] [2] [3] [4] [5] [6] [7] [8] [9] -
Schafer, A. G.; Wieting, J. M.; Mattson, A. E. Org. Lett. 2011, 13, 5228–5231. doi:10.1021/ol2021115
Return to citation in text: [1] [2] -
Wieting, J. M.; Fisher, T. J.; Schafer, A. G.; Visco, M. D.; Gallucci, J. C.; Mattson, A. E. Eur. J. Org. Chem. 2015, 525–533. doi:10.1002/ejoc.201403441
Return to citation in text: [1] [2] [3] [4] [5] [6] [7] -
Hardman-Baldwin, A. M.; Visco, M. D.; Wieting, J. M.; Stern, C.; Kondo, S.-i.; Mattson, A. E. Org. Lett. 2016, 18, 3766–3769. doi:10.1021/acs.orglett.6b01783
Return to citation in text: [1] [2] [3] [4] -
Kondo, S.-i.; Bie, Y.; Yamamura, M. Org. Lett. 2013, 15, 520–523. doi:10.1021/ol303332k
Return to citation in text: [1] -
Schott, G.; Engelbrecht, L.; Holdt, H. J. Z. Anorg. Allg. Chem. 1979, 459, 177–186. doi:10.1002/zaac.19794590119
Return to citation in text: [1] [2] [3] -
Beckmann, J.; Dakternieks, D.; Duthie, A.; Larchin, M. L.; Tiekink, E. R. T. Appl. Organomet. Chem. 2003, 17, 52–62. doi:10.1002/aoc.380
Return to citation in text: [1] [2] [3] -
Alpagut, Y.; Goldfuss, B.; Neudörfl, J.-M. Beilstein J. Org. Chem. 2008, 4, No. 25. doi:10.3762/bjoc.4.25
Return to citation in text: [1] [2] [3] [4] -
Kop-Weiershausen, T.; Lex, J.; Neudörfl, J.-M.; Goldfuss, B. Beilstein J. Org. Chem. 2005, 1, No. 6. doi:10.1186/1860-5397-1-6
Return to citation in text: [1] [2] [3] -
Lange, D. A.; Neudörfl, J.-M.; Goldfuss, B. Tetrahedron 2006, 62, 3704–3709. doi:10.1016/j.tet.2006.01.060
Return to citation in text: [1] [2] [3] -
Goldfuss, B.; Löschmann, T.; Kop-Weiershausen, T.; Neudörfl, J.; Rominger, F. Beilstein J. Org. Chem. 2006, 2, No. 7. doi:10.1186/1860-5397-2-7
Return to citation in text: [1] -
Trillo, R. B.; Leven, M.; Neudörfl, J. M.; Goldfuss, B. Adv. Synth. Catal. 2012, 354, 1451–1465. doi:10.1002/adsc.201100924
Return to citation in text: [1] -
Soki, F.; Neudörfl, J.-M.; Goldfuss, B. J. Organomet. Chem. 2008, 693, 2139–2146. doi:10.1016/j.jorganchem.2008.03.013
Return to citation in text: [1] -
Blanco Trillo, R.; Neudörfl, J. M.; Goldfuss, B. Beilstein J. Org. Chem. 2015, 11, 313–322. doi:10.3762/bjoc.11.36
Return to citation in text: [1] -
Schubert, U.; Neugebauer, W.; von Ragué Schleyer, P. J. Chem. Soc., Chem. Commun. 1982, 1184–1185. doi:10.1039/c39820001184
Return to citation in text: [1] -
Spirk, S.; Nieger, M.; Rechberger, G. N.; Pietschnig, R. Appl. Organomet. Chem. 2006, 20, 683–686. doi:10.1002/aoc.1117
Return to citation in text: [1] [2] [3] [4] -
Schäfer, A.; Weidenbruch, M.; Pohl, S.; Saak, W. Z. Naturforsch., B: J. Chem. Sci. 1990, 45, 1363–1368. doi:10.1515/znb-1990-1004
Return to citation in text: [1] -
Bento, A. P.; Bickelhaupt, F. M. J. Org. Chem. 2007, 72, 2201–2207. doi:10.1021/jo070076e
Return to citation in text: [1] [2] -
Hamlin, T. A.; van Beek, B.; Wolters, L. P.; Bickelhaupt, F. M. Chem. – Eur. J. 2018, 24, 5927–5938. doi:10.1002/chem.201706075
Return to citation in text: [1] [2] -
Hupf, E.; Olaru, M.; Raţ, C. I.; Fugel, M.; Hübschle, C. B.; Lork, E.; Grabowsky, S.; Mebs, S.; Beckmann, J. Chem. – Eur. J. 2017, 23, 10568–10579. doi:10.1002/chem.201700992
Return to citation in text: [1] [2] -
Ignatov, S. K.; Sennikov, P. G.; Razuvaev, A. G.; Chuprov, L. A.; Schrems, O.; Ault, B. S. J. Phys. Chem. A 2003, 107, 8705–8713. doi:10.1021/jp034618h
Return to citation in text: [1] [2] [3] -
Ignatyev, I. S.; Schaefer, H. F. Organometallics 2001, 20, 3113–3121. doi:10.1021/om010181t
Return to citation in text: [1] [2] [3] -
Chipanina, N. N.; Lazareva, N. F.; Oznobikhina, L. P.; Lazarev, I. M.; Shainyan, B. A. Comput. Theor. Chem. 2015, 1070, 162–173. doi:10.1016/j.comptc.2015.08.008
Return to citation in text: [1] [2] [3] -
Matsubara, T.; Ito, T. J. Phys. Chem. A 2016, 120, 2636–2646. doi:10.1021/acs.jpca.6b02308
Return to citation in text: [1] [2] -
Cossi, M.; Barone, V.; Cammi, R.; Tomasi, J. Chem. Phys. Lett. 1996, 255, 327–335. doi:10.1016/0009-2614(96)00349-1
Return to citation in text: [1] -
Mennucci, B.; Cancès, E.; Tomasi, J. J. Phys. Chem. B 1997, 101, 10506–10517. doi:10.1021/jp971959k
Return to citation in text: [1] -
Koch, W.; Holthausen, M. C. A chemist's guide to density functional theory, 5. reprint, 2nd ed.; Wiley-VCH: Weinheim, 2008.
Return to citation in text: [1] -
Grunenberg, J. Chem. Sci. 2015, 6, 4086–4088. doi:10.1039/c5sc01322d
Return to citation in text: [1] -
West, R.; Baney, R. H. J. Am. Chem. Soc. 1959, 81, 6145–6148. doi:10.1021/ja01532a011
Return to citation in text: [1] -
Steiner, T. Angew. Chem., Int. Ed. 2002, 41, 48–76. doi:10.1002/1521-3773(20020104)41:1<48::aid-anie48>3.0.co;2-u
Return to citation in text: [1] -
Thordarson, P. Chem. Soc. Rev. 2011, 40, 1305–1323. doi:10.1039/c0cs00062k
Return to citation in text: [1] [2] [3] -
Taylor, M. S.; Tokunaga, N.; Jacobsen, E. N. Angew. Chem., Int. Ed. 2005, 44, 6700–6704. doi:10.1002/anie.200502277
Return to citation in text: [1] [2] [3] -
Reisman, S. E.; Doyle, A. G.; Jacobsen, E. N. J. Am. Chem. Soc. 2008, 130, 7198–7199. doi:10.1021/ja801514m
Return to citation in text: [1] [2] -
Berkessel, A.; Das, S.; Pekel, D.; Neudörfl, J.-M. Angew. Chem., Int. Ed. 2014, 53, 11660–11664. doi:10.1002/anie.201403778
Return to citation in text: [1] -
Gaussian 09, Rev. D.01; Gaussian, Inc.: Wallingford CT, 2013.
Return to citation in text: [1] -
NIST Computational Chemistry Comparison and Benchmark Database. NIST Standard Reference Database Number 101. http://cccbdb.nist.gov/.
Return to citation in text: [1]
55. | Goldfuss, B.; Löschmann, T.; Kop-Weiershausen, T.; Neudörfl, J.; Rominger, F. Beilstein J. Org. Chem. 2006, 2, No. 7. doi:10.1186/1860-5397-2-7 |
56. | Trillo, R. B.; Leven, M.; Neudörfl, J. M.; Goldfuss, B. Adv. Synth. Catal. 2012, 354, 1451–1465. doi:10.1002/adsc.201100924 |
57. | Soki, F.; Neudörfl, J.-M.; Goldfuss, B. J. Organomet. Chem. 2008, 693, 2139–2146. doi:10.1016/j.jorganchem.2008.03.013 |
58. | Blanco Trillo, R.; Neudörfl, J. M.; Goldfuss, B. Beilstein J. Org. Chem. 2015, 11, 313–322. doi:10.3762/bjoc.11.36 |
60. | Spirk, S.; Nieger, M.; Rechberger, G. N.; Pietschnig, R. Appl. Organomet. Chem. 2006, 20, 683–686. doi:10.1002/aoc.1117 |
61. | Schäfer, A.; Weidenbruch, M.; Pohl, S.; Saak, W. Z. Naturforsch., B: J. Chem. Sci. 1990, 45, 1363–1368. doi:10.1515/znb-1990-1004 |
50. | Schott, G.; Engelbrecht, L.; Holdt, H. J. Z. Anorg. Allg. Chem. 1979, 459, 177–186. doi:10.1002/zaac.19794590119 |
51. | Beckmann, J.; Dakternieks, D.; Duthie, A.; Larchin, M. L.; Tiekink, E. R. T. Appl. Organomet. Chem. 2003, 17, 52–62. doi:10.1002/aoc.380 |
50. | Schott, G.; Engelbrecht, L.; Holdt, H. J. Z. Anorg. Allg. Chem. 1979, 459, 177–186. doi:10.1002/zaac.19794590119 |
39. | Kondo, S.-i.; Harada, T.; Tanaka, R.; Unno, M. Org. Lett. 2006, 8, 4621–4624. doi:10.1021/ol061822p |
52. | Alpagut, Y.; Goldfuss, B.; Neudörfl, J.-M. Beilstein J. Org. Chem. 2008, 4, No. 25. doi:10.3762/bjoc.4.25 |
54. | Lange, D. A.; Neudörfl, J.-M.; Goldfuss, B. Tetrahedron 2006, 62, 3704–3709. doi:10.1016/j.tet.2006.01.060 |
53. | Kop-Weiershausen, T.; Lex, J.; Neudörfl, J.-M.; Goldfuss, B. Beilstein J. Org. Chem. 2005, 1, No. 6. doi:10.1186/1860-5397-1-6 |
40. | Liu, M.; Tran, N. T.; Franz, A. K.; Lee, J. K. J. Org. Chem. 2011, 76, 7186–7194. doi:10.1021/jo201214x |
28. | Auvil, T. J.; Schafer, A. G.; Mattson, A. E. Eur. J. Org. Chem. 2014, 2633–2646. doi:10.1002/ejoc.201400035 |
52. | Alpagut, Y.; Goldfuss, B.; Neudörfl, J.-M. Beilstein J. Org. Chem. 2008, 4, No. 25. doi:10.3762/bjoc.4.25 |
54. | Lange, D. A.; Neudörfl, J.-M.; Goldfuss, B. Tetrahedron 2006, 62, 3704–3709. doi:10.1016/j.tet.2006.01.060 |
59. | Schubert, U.; Neugebauer, W.; von Ragué Schleyer, P. J. Chem. Soc., Chem. Commun. 1982, 1184–1185. doi:10.1039/c39820001184 |
51. | Beckmann, J.; Dakternieks, D.; Duthie, A.; Larchin, M. L.; Tiekink, E. R. T. Appl. Organomet. Chem. 2003, 17, 52–62. doi:10.1002/aoc.380 |
60. | Spirk, S.; Nieger, M.; Rechberger, G. N.; Pietschnig, R. Appl. Organomet. Chem. 2006, 20, 683–686. doi:10.1002/aoc.1117 |
60. | Spirk, S.; Nieger, M.; Rechberger, G. N.; Pietschnig, R. Appl. Organomet. Chem. 2006, 20, 683–686. doi:10.1002/aoc.1117 |
60. | Spirk, S.; Nieger, M.; Rechberger, G. N.; Pietschnig, R. Appl. Organomet. Chem. 2006, 20, 683–686. doi:10.1002/aoc.1117 |
73. | West, R.; Baney, R. H. J. Am. Chem. Soc. 1959, 81, 6145–6148. doi:10.1021/ja01532a011 |
41. | Tran, N. T.; Min, T.; Franz, A. K. Chem. – Eur. J. 2011, 17, 9897–9900. doi:10.1002/chem.201101492 |
42. | Tran, N. T.; Wilson, S. O.; Franz, A. K. Org. Lett. 2012, 14, 186–189. doi:10.1021/ol202971m |
43. | Tran, N. T.; Wilson, S. O.; Franz, A. K. Chem. Commun. 2014, 50, 3738–3740. doi:10.1039/c4cc00672k |
44. | Wilson, S. O.; Tran, N. T.; Franz, A. K. Organometallics 2012, 31, 6715–6718. doi:10.1021/om300736n |
71. | Koch, W.; Holthausen, M. C. A chemist's guide to density functional theory, 5. reprint, 2nd ed.; Wiley-VCH: Weinheim, 2008. |
62. | Bento, A. P.; Bickelhaupt, F. M. J. Org. Chem. 2007, 72, 2201–2207. doi:10.1021/jo070076e |
63. | Hamlin, T. A.; van Beek, B.; Wolters, L. P.; Bickelhaupt, F. M. Chem. – Eur. J. 2018, 24, 5927–5938. doi:10.1002/chem.201706075 |
64. | Hupf, E.; Olaru, M.; Raţ, C. I.; Fugel, M.; Hübschle, C. B.; Lork, E.; Grabowsky, S.; Mebs, S.; Beckmann, J. Chem. – Eur. J. 2017, 23, 10568–10579. doi:10.1002/chem.201700992 |
65. | Ignatov, S. K.; Sennikov, P. G.; Razuvaev, A. G.; Chuprov, L. A.; Schrems, O.; Ault, B. S. J. Phys. Chem. A 2003, 107, 8705–8713. doi:10.1021/jp034618h |
66. | Ignatyev, I. S.; Schaefer, H. F. Organometallics 2001, 20, 3113–3121. doi:10.1021/om010181t |
67. | Chipanina, N. N.; Lazareva, N. F.; Oznobikhina, L. P.; Lazarev, I. M.; Shainyan, B. A. Comput. Theor. Chem. 2015, 1070, 162–173. doi:10.1016/j.comptc.2015.08.008 |
68. | Matsubara, T.; Ito, T. J. Phys. Chem. A 2016, 120, 2636–2646. doi:10.1021/acs.jpca.6b02308 |
69. | Cossi, M.; Barone, V.; Cammi, R.; Tomasi, J. Chem. Phys. Lett. 1996, 255, 327–335. doi:10.1016/0009-2614(96)00349-1 |
70. | Mennucci, B.; Cancès, E.; Tomasi, J. J. Phys. Chem. B 1997, 101, 10506–10517. doi:10.1021/jp971959k |
62. | Bento, A. P.; Bickelhaupt, F. M. J. Org. Chem. 2007, 72, 2201–2207. doi:10.1021/jo070076e |
63. | Hamlin, T. A.; van Beek, B.; Wolters, L. P.; Bickelhaupt, F. M. Chem. – Eur. J. 2018, 24, 5927–5938. doi:10.1002/chem.201706075 |
64. | Hupf, E.; Olaru, M.; Raţ, C. I.; Fugel, M.; Hübschle, C. B.; Lork, E.; Grabowsky, S.; Mebs, S.; Beckmann, J. Chem. – Eur. J. 2017, 23, 10568–10579. doi:10.1002/chem.201700992 |
65. | Ignatov, S. K.; Sennikov, P. G.; Razuvaev, A. G.; Chuprov, L. A.; Schrems, O.; Ault, B. S. J. Phys. Chem. A 2003, 107, 8705–8713. doi:10.1021/jp034618h |
66. | Ignatyev, I. S.; Schaefer, H. F. Organometallics 2001, 20, 3113–3121. doi:10.1021/om010181t |
67. | Chipanina, N. N.; Lazareva, N. F.; Oznobikhina, L. P.; Lazarev, I. M.; Shainyan, B. A. Comput. Theor. Chem. 2015, 1070, 162–173. doi:10.1016/j.comptc.2015.08.008 |
68. | Matsubara, T.; Ito, T. J. Phys. Chem. A 2016, 120, 2636–2646. doi:10.1021/acs.jpca.6b02308 |
65. | Ignatov, S. K.; Sennikov, P. G.; Razuvaev, A. G.; Chuprov, L. A.; Schrems, O.; Ault, B. S. J. Phys. Chem. A 2003, 107, 8705–8713. doi:10.1021/jp034618h |
66. | Ignatyev, I. S.; Schaefer, H. F. Organometallics 2001, 20, 3113–3121. doi:10.1021/om010181t |
67. | Chipanina, N. N.; Lazareva, N. F.; Oznobikhina, L. P.; Lazarev, I. M.; Shainyan, B. A. Comput. Theor. Chem. 2015, 1070, 162–173. doi:10.1016/j.comptc.2015.08.008 |
74. | Steiner, T. Angew. Chem., Int. Ed. 2002, 41, 48–76. doi:10.1002/1521-3773(20020104)41:1<48::aid-anie48>3.0.co;2-u |
41. | Tran, N. T.; Min, T.; Franz, A. K. Chem. – Eur. J. 2011, 17, 9897–9900. doi:10.1002/chem.201101492 |
42. | Tran, N. T.; Wilson, S. O.; Franz, A. K. Org. Lett. 2012, 14, 186–189. doi:10.1021/ol202971m |
43. | Tran, N. T.; Wilson, S. O.; Franz, A. K. Chem. Commun. 2014, 50, 3738–3740. doi:10.1039/c4cc00672k |
44. | Wilson, S. O.; Tran, N. T.; Franz, A. K. Organometallics 2012, 31, 6715–6718. doi:10.1021/om300736n |
41. | Tran, N. T.; Min, T.; Franz, A. K. Chem. – Eur. J. 2011, 17, 9897–9900. doi:10.1002/chem.201101492 |
42. | Tran, N. T.; Wilson, S. O.; Franz, A. K. Org. Lett. 2012, 14, 186–189. doi:10.1021/ol202971m |
43. | Tran, N. T.; Wilson, S. O.; Franz, A. K. Chem. Commun. 2014, 50, 3738–3740. doi:10.1039/c4cc00672k |
44. | Wilson, S. O.; Tran, N. T.; Franz, A. K. Organometallics 2012, 31, 6715–6718. doi:10.1021/om300736n |
1. | Chandrasekhar, V.; Boomishankar, R.; Nagendran, S. Chem. Rev. 2004, 104, 5847–5910. doi:10.1021/cr0306135 |
2. | Hernández, D.; Mose, R.; Skrydstrup, T. Org. Lett. 2011, 13, 732–735. doi:10.1021/ol102968g |
3. | Kim, J. K.; Sieburth, S. M. J. Org. Chem. 2012, 77, 2901–2906. doi:10.1021/jo300175t |
4. | Madsen, J. L. H.; Andersen, T. L.; Santamaria, S.; Nagase, H.; Enghild, J. J.; Skrydstrup, T. J. Med. Chem. 2012, 55, 7900–7908. doi:10.1021/jm301000k |
5. | Guan, Y.; Attard, J. W.; Visco, M. D.; Fisher, T. J.; Mattson, A. E. Chem. – Eur. J. 2018, 24, 7123–7127. doi:10.1002/chem.201801304 |
6. | Visco, M. D.; Attard, J.; Guan, Y.; Mattson, A. E. Tetrahedron Lett. 2017, 58, 2623–2628. doi:10.1016/j.tetlet.2017.05.045 |
23. | Shaffer, L. H.; Flanigen, E. M. J. Phys. Chem. 1957, 61, 1591–1595. doi:10.1021/j150558a004 |
24. | Shaffer, L. H.; Flanigen, E. M. J. Phys. Chem. 1957, 61, 1595–1600. doi:10.1021/j150558a005 |
25. | Swain, C. G.; Esteve, R. M.; Jones, R. H. J. Am. Chem. Soc. 1949, 71, 965–971. doi:10.1021/ja01171a056 |
40. | Liu, M.; Tran, N. T.; Franz, A. K.; Lee, J. K. J. Org. Chem. 2011, 76, 7186–7194. doi:10.1021/jo201214x |
41. | Tran, N. T.; Min, T.; Franz, A. K. Chem. – Eur. J. 2011, 17, 9897–9900. doi:10.1002/chem.201101492 |
42. | Tran, N. T.; Wilson, S. O.; Franz, A. K. Org. Lett. 2012, 14, 186–189. doi:10.1021/ol202971m |
43. | Tran, N. T.; Wilson, S. O.; Franz, A. K. Chem. Commun. 2014, 50, 3738–3740. doi:10.1039/c4cc00672k |
44. | Wilson, S. O.; Tran, N. T.; Franz, A. K. Organometallics 2012, 31, 6715–6718. doi:10.1021/om300736n |
45. | Schafer, A. G.; Wieting, J. M.; Fisher, T. J.; Mattson, A. E. Angew. Chem., Int. Ed. 2013, 52, 11321–11324. doi:10.1002/anie.201305496 |
47. | Wieting, J. M.; Fisher, T. J.; Schafer, A. G.; Visco, M. D.; Gallucci, J. C.; Mattson, A. E. Eur. J. Org. Chem. 2015, 525–533. doi:10.1002/ejoc.201403441 |
18. | Safa, K. D.; Asadi, A.; Sargordan, M. J. Organomet. Chem. 1997, 545–546, 61–65. doi:10.1016/s0022-328x(97)00302-1 |
19. | George, P. D.; Sommer, L. H.; Whitmore, F. C. J. Am. Chem. Soc. 1953, 75, 1585–1588. doi:10.1021/ja01103a020 |
20. | Cusa, N. W.; Kipping, F. S. J. Chem. Soc. 1932, 2205–2209. doi:10.1039/jr9320002205 |
21. | Ojima, I.; Fuchikami, T.; Yatabe, M. J. Organomet. Chem. 1984, 260, 335–346. doi:10.1016/s0022-328x(00)99483-x |
22. | Robinson, R.; Kipping, F. S. J. Chem. Soc., Trans. 1912, 101, 2142–2155. doi:10.1039/ct9120102142 |
45. | Schafer, A. G.; Wieting, J. M.; Fisher, T. J.; Mattson, A. E. Angew. Chem., Int. Ed. 2013, 52, 11321–11324. doi:10.1002/anie.201305496 |
46. | Schafer, A. G.; Wieting, J. M.; Mattson, A. E. Org. Lett. 2011, 13, 5228–5231. doi:10.1021/ol2021115 |
47. | Wieting, J. M.; Fisher, T. J.; Schafer, A. G.; Visco, M. D.; Gallucci, J. C.; Mattson, A. E. Eur. J. Org. Chem. 2015, 525–533. doi:10.1002/ejoc.201403441 |
48. | Hardman-Baldwin, A. M.; Visco, M. D.; Wieting, J. M.; Stern, C.; Kondo, S.-i.; Mattson, A. E. Org. Lett. 2016, 18, 3766–3769. doi:10.1021/acs.orglett.6b01783 |
49. | Kondo, S.-i.; Bie, Y.; Yamamura, M. Org. Lett. 2013, 15, 520–523. doi:10.1021/ol303332k |
12. | Carilla, J.; Fajarí, L.; Juliá, L.; Riera, J.; Lloveras, J.; Verdaguer, N.; Fita, I. J. Organomet. Chem. 1992, 423, 163–171. doi:10.1016/0022-328x(92)83111-t |
13. | Bruña, S.; Garrido-Castro, A. F.; Perles, J.; Montero-Campillo, M. M.; Mó, O.; Kaifer, A. E.; Cuadrado, I. Organometallics 2016, 35, 3507–3519. doi:10.1021/acs.organomet.6b00559 |
14. | Auner, N.; Probst, R.; Heikenwälder, C.-R.; Herdtweck, E.; Gamper, S.; Müller, G. Z. Naturforsch., B: J. Chem. Sci. 1993, 48, 1625–1634. doi:10.1515/znb-1993-1123 |
15. | Belzner, J.; Schär, D.; Herbst-Irmer, R.; Kneisel, B. O.; Noltemeyer, M. Tetrahedron 1998, 54, 8481–8500. doi:10.1016/s0040-4020(98)00451-7 |
16. | Hurkes, N.; Belaj, F.; Koe, J. R.; Pietschnig, R. Appl. Organomet. Chem. 2018, 32, e4427. doi:10.1002/aoc.4427 |
17. | Shamaev, A. E.; Glazkov, A. A.; Ignatenko, A. V.; Sakharov, A. M. Russ. Chem. Bull. 2005, 54, 1250–1253. doi:10.1007/s11172-005-0389-y |
6. | Visco, M. D.; Attard, J.; Guan, Y.; Mattson, A. E. Tetrahedron Lett. 2017, 58, 2623–2628. doi:10.1016/j.tetlet.2017.05.045 |
38. | Brak, K.; Jacobsen, E. N. Angew. Chem., Int. Ed. 2013, 52, 534–561. doi:10.1002/anie.201205449 |
2. | Hernández, D.; Mose, R.; Skrydstrup, T. Org. Lett. 2011, 13, 732–735. doi:10.1021/ol102968g |
4. | Madsen, J. L. H.; Andersen, T. L.; Santamaria, S.; Nagase, H.; Enghild, J. J.; Skrydstrup, T. J. Med. Chem. 2012, 55, 7900–7908. doi:10.1021/jm301000k |
7. | Plunkett, M. J.; Ellman, J. A. J. Org. Chem. 1995, 60, 6006–6007. doi:10.1021/jo00124a005 |
8. | Anderson, T. F.; Statham, M. A. J.; Carroll, M. A. Tetrahedron Lett. 2006, 47, 3353–3355. doi:10.1016/j.tetlet.2006.03.095 |
9. | Glekas, A.; Sieburth, S. M. Tetrahedron Lett. 2001, 42, 3799–3801. doi:10.1016/s0040-4039(01)00576-7 |
10. | Duong, H. Q.; Sieburth, S. M. J. Org. Chem. 2018, 83, 5398–5409. doi:10.1021/acs.joc.8b00116 |
11. | Yao, C.; Yu, J.; Wang, Y.; Tang, C.; Huang, C. Dent. Mater. 2018, 34, 809–818. doi:10.1016/j.dental.2018.02.004 |
39. | Kondo, S.-i.; Harada, T.; Tanaka, R.; Unno, M. Org. Lett. 2006, 8, 4621–4624. doi:10.1021/ol061822p |
45. | Schafer, A. G.; Wieting, J. M.; Fisher, T. J.; Mattson, A. E. Angew. Chem., Int. Ed. 2013, 52, 11321–11324. doi:10.1002/anie.201305496 |
47. | Wieting, J. M.; Fisher, T. J.; Schafer, A. G.; Visco, M. D.; Gallucci, J. C.; Mattson, A. E. Eur. J. Org. Chem. 2015, 525–533. doi:10.1002/ejoc.201403441 |
27. | Taylor, M. S.; Jacobsen, E. N. Angew. Chem., Int. Ed. 2006, 45, 1520–1543. doi:10.1002/anie.200503132 |
1. | Chandrasekhar, V.; Boomishankar, R.; Nagendran, S. Chem. Rev. 2004, 104, 5847–5910. doi:10.1021/cr0306135 |
28. | Auvil, T. J.; Schafer, A. G.; Mattson, A. E. Eur. J. Org. Chem. 2014, 2633–2646. doi:10.1002/ejoc.201400035 |
36. | Kondo, S.-i.; Okada, N.; Tanaka, R.; Yamamura, M.; Unno, M. Tetrahedron Lett. 2009, 50, 2754–2757. doi:10.1016/j.tetlet.2009.03.134 |
47. | Wieting, J. M.; Fisher, T. J.; Schafer, A. G.; Visco, M. D.; Gallucci, J. C.; Mattson, A. E. Eur. J. Org. Chem. 2015, 525–533. doi:10.1002/ejoc.201403441 |
75. | Thordarson, P. Chem. Soc. Rev. 2011, 40, 1305–1323. doi:10.1039/c0cs00062k |
30. | Zhang, Z.; Schreiner, P. R. Chem. Soc. Rev. 2009, 38, 1187–1198. doi:10.1039/b801793j |
31. | Takemoto, Y. Chem. Pharm. Bull. 2010, 58, 593–601. doi:10.1248/cpb.58.593 |
37. | Busschaert, N.; Caltagirone, C.; Van Rossom, W.; Gale, P. A. Chem. Rev. 2015, 115, 8038–8155. doi:10.1021/acs.chemrev.5b00099 |
29. | Alemán, J.; Parra, A.; Jiang, H.; Jørgensen, K. A. Chem. – Eur. J. 2011, 17, 6890–6899. doi:10.1002/chem.201003694 |
43. | Tran, N. T.; Wilson, S. O.; Franz, A. K. Chem. Commun. 2014, 50, 3738–3740. doi:10.1039/c4cc00672k |
26. | Doyle, A. G.; Jacobsen, E. N. Chem. Rev. 2007, 107, 5713–5743. doi:10.1021/cr068373r |
27. | Taylor, M. S.; Jacobsen, E. N. Angew. Chem., Int. Ed. 2006, 45, 1520–1543. doi:10.1002/anie.200503132 |
28. | Auvil, T. J.; Schafer, A. G.; Mattson, A. E. Eur. J. Org. Chem. 2014, 2633–2646. doi:10.1002/ejoc.201400035 |
32. | Klare, H.; Neudörfl, J. M.; Goldfuss, B. Beilstein J. Org. Chem. 2014, 10, 224–236. doi:10.3762/bjoc.10.18 |
33. | Klare, H.; Hanft, S.; Neudörfl, J. M.; Schlörer, N. E.; Griesbeck, A.; Goldfuss, B. Chem. – Eur. J. 2014, 20, 11847–11855. doi:10.1002/chem.201403013 |
34. | Wolf, F. F.; Klare, H.; Goldfuss, B. J. Org. Chem. 2016, 81, 1762–1768. doi:10.1021/acs.joc.5b02167 |
35. | Wolf, F. F.; Neudörfl, J.-M.; Goldfuss, B. New J. Chem. 2018, 42, 4854–4870. doi:10.1039/c7nj04660j |
43. | Tran, N. T.; Wilson, S. O.; Franz, A. K. Chem. Commun. 2014, 50, 3738–3740. doi:10.1039/c4cc00672k |
39. | Kondo, S.-i.; Harada, T.; Tanaka, R.; Unno, M. Org. Lett. 2006, 8, 4621–4624. doi:10.1021/ol061822p |
47. | Wieting, J. M.; Fisher, T. J.; Schafer, A. G.; Visco, M. D.; Gallucci, J. C.; Mattson, A. E. Eur. J. Org. Chem. 2015, 525–533. doi:10.1002/ejoc.201403441 |
50. | Schott, G.; Engelbrecht, L.; Holdt, H. J. Z. Anorg. Allg. Chem. 1979, 459, 177–186. doi:10.1002/zaac.19794590119 |
51. | Beckmann, J.; Dakternieks, D.; Duthie, A.; Larchin, M. L.; Tiekink, E. R. T. Appl. Organomet. Chem. 2003, 17, 52–62. doi:10.1002/aoc.380 |
45. | Schafer, A. G.; Wieting, J. M.; Fisher, T. J.; Mattson, A. E. Angew. Chem., Int. Ed. 2013, 52, 11321–11324. doi:10.1002/anie.201305496 |
76. | Taylor, M. S.; Tokunaga, N.; Jacobsen, E. N. Angew. Chem., Int. Ed. 2005, 44, 6700–6704. doi:10.1002/anie.200502277 |
45. | Schafer, A. G.; Wieting, J. M.; Fisher, T. J.; Mattson, A. E. Angew. Chem., Int. Ed. 2013, 52, 11321–11324. doi:10.1002/anie.201305496 |
76. | Taylor, M. S.; Tokunaga, N.; Jacobsen, E. N. Angew. Chem., Int. Ed. 2005, 44, 6700–6704. doi:10.1002/anie.200502277 |
45. | Schafer, A. G.; Wieting, J. M.; Fisher, T. J.; Mattson, A. E. Angew. Chem., Int. Ed. 2013, 52, 11321–11324. doi:10.1002/anie.201305496 |
45. | Schafer, A. G.; Wieting, J. M.; Fisher, T. J.; Mattson, A. E. Angew. Chem., Int. Ed. 2013, 52, 11321–11324. doi:10.1002/anie.201305496 |
76. | Taylor, M. S.; Tokunaga, N.; Jacobsen, E. N. Angew. Chem., Int. Ed. 2005, 44, 6700–6704. doi:10.1002/anie.200502277 |
52. | Alpagut, Y.; Goldfuss, B.; Neudörfl, J.-M. Beilstein J. Org. Chem. 2008, 4, No. 25. doi:10.3762/bjoc.4.25 |
54. | Lange, D. A.; Neudörfl, J.-M.; Goldfuss, B. Tetrahedron 2006, 62, 3704–3709. doi:10.1016/j.tet.2006.01.060 |
53. | Kop-Weiershausen, T.; Lex, J.; Neudörfl, J.-M.; Goldfuss, B. Beilstein J. Org. Chem. 2005, 1, No. 6. doi:10.1186/1860-5397-1-6 |
53. | Kop-Weiershausen, T.; Lex, J.; Neudörfl, J.-M.; Goldfuss, B. Beilstein J. Org. Chem. 2005, 1, No. 6. doi:10.1186/1860-5397-1-6 |
45. | Schafer, A. G.; Wieting, J. M.; Fisher, T. J.; Mattson, A. E. Angew. Chem., Int. Ed. 2013, 52, 11321–11324. doi:10.1002/anie.201305496 |
47. | Wieting, J. M.; Fisher, T. J.; Schafer, A. G.; Visco, M. D.; Gallucci, J. C.; Mattson, A. E. Eur. J. Org. Chem. 2015, 525–533. doi:10.1002/ejoc.201403441 |
80. | NIST Computational Chemistry Comparison and Benchmark Database. NIST Standard Reference Database Number 101. http://cccbdb.nist.gov/. |
47. | Wieting, J. M.; Fisher, T. J.; Schafer, A. G.; Visco, M. D.; Gallucci, J. C.; Mattson, A. E. Eur. J. Org. Chem. 2015, 525–533. doi:10.1002/ejoc.201403441 |
48. | Hardman-Baldwin, A. M.; Visco, M. D.; Wieting, J. M.; Stern, C.; Kondo, S.-i.; Mattson, A. E. Org. Lett. 2016, 18, 3766–3769. doi:10.1021/acs.orglett.6b01783 |
48. | Hardman-Baldwin, A. M.; Visco, M. D.; Wieting, J. M.; Stern, C.; Kondo, S.-i.; Mattson, A. E. Org. Lett. 2016, 18, 3766–3769. doi:10.1021/acs.orglett.6b01783 |
52. | Alpagut, Y.; Goldfuss, B.; Neudörfl, J.-M. Beilstein J. Org. Chem. 2008, 4, No. 25. doi:10.3762/bjoc.4.25 |
48. | Hardman-Baldwin, A. M.; Visco, M. D.; Wieting, J. M.; Stern, C.; Kondo, S.-i.; Mattson, A. E. Org. Lett. 2016, 18, 3766–3769. doi:10.1021/acs.orglett.6b01783 |
41. | Tran, N. T.; Min, T.; Franz, A. K. Chem. – Eur. J. 2011, 17, 9897–9900. doi:10.1002/chem.201101492 |
42. | Tran, N. T.; Wilson, S. O.; Franz, A. K. Org. Lett. 2012, 14, 186–189. doi:10.1021/ol202971m |
43. | Tran, N. T.; Wilson, S. O.; Franz, A. K. Chem. Commun. 2014, 50, 3738–3740. doi:10.1039/c4cc00672k |
77. | Reisman, S. E.; Doyle, A. G.; Jacobsen, E. N. J. Am. Chem. Soc. 2008, 130, 7198–7199. doi:10.1021/ja801514m |
78. | Berkessel, A.; Das, S.; Pekel, D.; Neudörfl, J.-M. Angew. Chem., Int. Ed. 2014, 53, 11660–11664. doi:10.1002/anie.201403778 |
45. | Schafer, A. G.; Wieting, J. M.; Fisher, T. J.; Mattson, A. E. Angew. Chem., Int. Ed. 2013, 52, 11321–11324. doi:10.1002/anie.201305496 |
46. | Schafer, A. G.; Wieting, J. M.; Mattson, A. E. Org. Lett. 2011, 13, 5228–5231. doi:10.1021/ol2021115 |
77. | Reisman, S. E.; Doyle, A. G.; Jacobsen, E. N. J. Am. Chem. Soc. 2008, 130, 7198–7199. doi:10.1021/ja801514m |
© 2019 Fox et al.; licensee Beilstein-Institut.
This is an Open Access article under the terms of the Creative Commons Attribution License (http://creativecommons.org/licenses/by/4.0). Please note that the reuse, redistribution and reproduction in particular requires that the authors and source are credited.
The license is subject to the Beilstein Journal of Organic Chemistry terms and conditions: (https://www.beilstein-journals.org/bjoc)