Abstract
Secondary metabolites produced by actinomycete strains undoubtedly have great potential for use in applied research areas such as drug discovery. However, it is becoming difficult to obtain novel compounds because of repeated isolation around the world. Therefore, a new strategy for discovering novel secondary metabolites is needed. Many researchers believe that actinomycetes have as yet unanalyzed secondary metabolic activities, and the associated undiscovered secondary metabolite biosynthesis genes are called “silent” genes. This review outlines several approaches to further activate the metabolic potential of actinomycetes.
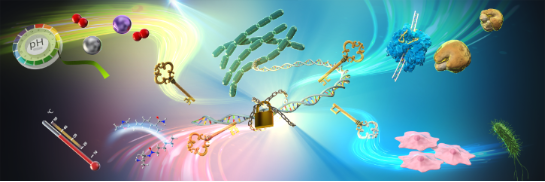
Graphical Abstract
Introduction
Research clearly indicates that compounds derived from natural products have contributed greatly to enhancing the welfare of human society. For example, the structures of compounds produced by microorganisms are very diverse, facilitating their application in the development of therapeutic drugs [1], agrochemicals [2], veterinary medicines [3], medium supplements for selective microbial/cell culture [4-6], food preservatives/colorings [7,8], and biochemical reagents for pharmacological/chemical biology studies [9]. This review has focused particularly on compounds produced by actinomycetes. Secondary metabolites produced by actinomycetes, representing a class of compounds with a diverse chemical space, have promising potential as sources of bioactive substances [10]. Due to his discovery of streptomycin from Streptomyces griseus, Waksman was awarded the Nobel Prize in Physiology and Medicine in 1952 [11]. Ōmura, who discovered avermectin from Streptomyces avermitilis, received the same award in 2015 [12]. While there are many such brilliant achievements, researchers are finding it increasingly difficult to isolate novel compounds from secondary metabolites produced by actinomycetes [13,14]. This is the result of more than 50 years of effort to scrutinize substances produced by actinomycetes. However, many researchers have questioned whether the potential secondary metabolic capacity of actinomycetes has been fully analyzed and have continued to search for compounds from actinomycetes based on original ideas. For example, in searching for compounds based on biological activity, a proper screening system is critical [15]. Screening systems are broadly classified as either forward screening (searching for compounds that induce phenotypic changes) or reverse screening (searching for compounds that act directly on specific molecules). Imoto and colleagues constructed a reverse screening system that targets a malignant factor of prostate cancer and thereby identified several novel compounds from actinomycete strains [16-19].
Many researchers have focused on identifying secondary metabolites from actinomycetes of the genus Streptomyces due to the prominence of species of this genus in surface soils and the difficulty of isolating members of other genera from natural environments. For this reason, actinomycetes other than those of the genus Streptomyces are referred to as “rare actinomycetes”, and the secondary metabolites produced by these species have thus attracted increasing attention. Indeed, an increasing proportion of newly identified antibiotics are reportedly produced by rare actinomycetes [20]. Igarashi and colleagues have also focused on various rare actinomycetes, analyzing their secondary metabolic activity and demonstrating the importance of studying new genera and species [21-25]. On the other hand, it is now thought that secondary metabolic activities remain to be analyzed in actinomycetes, including Streptomyces strains, which were considered to have been completely characterized. This may be because the number of discovered compounds is small compared to the number of secondary metabolite biosynthesis genes harbored by actinomycetes [26-28]. For example, in Streptomyces avermitilis, 38 secondary metabolite biosynthetic gene clusters have been identified by genome sequencing. Interestingly, only 16 secondary metabolites of S. avermitilis have been identified to date, even though the species is widely used as a standard actinomycete. This means that only 40% of the secondary metabolites from the 38 gene clusters have been identified. These as yet undiscovered secondary metabolite biosynthesis genes are called “silent genes”, because they are either not expressed or their expression levels are low under normal culture conditions. A number of studies have reported methods to activate these genes, and many new compounds have been discovered. In this review, we outline the silent gene activation methods, including the authors’ efforts (Figure 1).
![[1860-5397-20-69-1]](/bjoc/content/figures/1860-5397-20-69-1.png?scale=2.0&max-width=1024&background=FFFFFF)
Figure 1: Schematic diagram of methods to activate silent genes in actinomycetes as presented in this review. This figure was created by Science Graphics Co., Ltd. This content is not subject to CC BY 4.0.
Figure 1: Schematic diagram of methods to activate silent genes in actinomycetes as presented in this review....
Review
Artificial methods
Several target-oriented methods focusing on specific biosynthetic genes and regulatory factors and artificial methods have been reported to activate secondary metabolism in actinomycetes. The technique known as “heterologous expression”, in which a biosynthetic gene cluster of a secondary metabolite is introduced into another organism, is a useful method for producing silent secondary metabolites (Figure 2a). For example, the cryptic trans-acyltransferase polyketide synthase biosynthetic gene cluster sdl (80 kb) from Streptomyces sp. B59 was cloned and transferred into a heterologous host, Streptomyces albus J1074, resulting in the production of a class of polycyclic macrolide shuangdaolides A (1), B, and D and dumulmycin (2) [29]. Furthermore, genome mining of the marine actinomycete Streptomyces seoulensis A01 enabled the identification of a giant type I polyketide synthase gene cluster (asm) [30]. Heterologous expression of the cryptic asm cluster using a bacterial artificial chromosome vector in a heterologous host led to the production of ansaseomycins A (3) and B.
Figure 2: Structures of secondary metabolites obtained from actinomycetes using artificial methods.
Figure 2: Structures of secondary metabolites obtained from actinomycetes using artificial methods.
A number of factors associated with the biosynthetic gene clusters of secondary metabolites regulate their expression; thus, controlling these factors can also be used to activate secondary metabolism (Figure 2b). For example, the production of some secondary metabolites in actinomycetes is regulated by various bacterial hormones, such as A-factor (4) [31] and SCB1 (5) [32]. Normally, the tetR regulator negatively regulates the expression of biosynthetic genes in actinomycetes, but bacterial hormones release this repression [33,34]. A-factor, SCB1, and avenolide (6) induce the production of streptomycin (7), actinorhodin (8), and avermectin (9), respectively [35-37]. The production of these metabolites is regulated by the respective tetR regulators ArpA, ScbR, and AvaR1, respectively [38-40]. Therefore, increasing the levels of bacterial hormones present or disruption of the tetR regulator have been reported in several studies as methods to improve target-substance production capacity. Interestingly, Wilbanks et al. reported the structure–activity relationships of bacterial hormones in secondary metabolite biosynthesis by diversifiable synthesis [41]. The ability to synthesize stereochemically pure bacterial hormones would greatly expedite studies of these repressors and ultimately lead to the discovery of regulatory systems for many secondary metabolites.
Overexpression of transcription factors present in biosynthetic gene clusters can also be employed to increase the production of secondary metabolites. For example, overexpression of Streptomyces antibiotic regulatory protein (SARP) family transcriptional activators led to the discovery of many new secondary metabolites [42]. Du et al. searched for SARP family transcriptional activators from the draft genome of Streptomyces sp. MSC090213JE08, and constructed recombinant strains with forced expression of each of the seven SARP genes. When these were cultured in four different media and examined for metabolic profiles, a total of nine unknown metabolites were found in the four SARP-expressing strains under specific culture conditions. Du et al. identified a new compound (designated ishigamide (10)) as 3-([2E,4E,6E,8E]-13-hydroxytetradeca-2,4,6,8-tetraenamido)propanoic acid and elucidated the biosynthetic machinery (Figure 2c) [43-45]. Seo et al. synthesized ishigamide and determined its absolute configuration [46].
Finally, several studies have reported the modulation of secondary metabolic activation in actinomycetes using small molecules. For example, Ochi et al. proposed the “ribosome engineering” technique, in which actinomycetes are cultured with antibiotics to activate secondary metabolism by inducing resistance, and this approach has contributed to the isolation of many new compounds [47]. The ribosome engineering method is based on the principle that a mutation in the ribosomal S12 protein, which is associated with the acquisition of antibiotic resistance, activates secondary metabolism. The ribosomal S12 protein mutation results in increased expression of translation factors, which leads to enhanced protein synthesis in secondary metabolism [47]. Liu et al. reported activation of the production of bohemamines 11–13, bacterial alkaloids containing a pyrrolizidine core with two unusual methyl groups, using a ribosome engineering approach in Streptomyces sp. CB02009 (Figure 2d) [48].
Seyedsayamdost et al. developed the high-throughput elicitor screening (HiTES) approach, a forward chemical genetics method that identifies small-molecule inducers of silent natural products (Figure 2e) [49]. Han et al. applied a fluorescence-based DNA cleavage assay coupled with HiTES to Streptomyces clavuligerus and identified the steroid 11α-hydroxyprogesterone (14) as an effective elicitor and characterized 10 cryptic enediyne-derived natural products, designated clavulynes A (15) and B–J with unusual carbonate and terminal olefin functionalities [50]. Thus, artificial methods of genetic engineering and chemistry also play an important role in the identification of novel secondary metabolites.
Culture conditions
Medium composition, pH, oxygen supply, light
Simply modifying the culture conditions is an important means of activating silent genes in actinomycetes. Because the cultivation conditions play a key role in metabolite production by actinomycetes, changing these parameters can significantly alter secondary metabolite production patterns. For example, OSMAC (one strain-multi compound), developed by Zeeck and co-workers in the early 2000s, is a method in which the target bacteria are cultured under various conditions (medium composition, temperature, pH, oxygen supply, light quality and quantity, addition of precursors and enzyme inhibitors, etc.) and all metabolites obtained from them are analyzed (Figure 3a) [51]. Zeeck's group has successfully isolated metabolites consisting of diverse structural classes from only a few microbial species by their OSMAC approach [52-54]. By applying this method, Rateb et al. and Sproule et al. discovered 22-membered macrolactone polyketides designated chaxalactins A–C (16–18), and polycyclic polyether natural products designated terrosamycins A and B (19, 20), respectively [55,56]. Thus, the OSMAC strategy has been successfully applied over the last 10 years to generate new secondary metabolites from a single microbial strain.
Figure 3: Structures of secondary metabolites obtained from actinomycetes by adjusting culture conditions.
Figure 3: Structures of secondary metabolites obtained from actinomycetes by adjusting culture conditions.
Sensor proteins for medium components are also present in actinomycetes, and a regulatory system for secondary metabolism has been reported [57]. For example, Świątek et al. proposed that Rok7B7, a transcriptional regulator, acts as the central control protein for carbon control in Streptomyces [58,59]. Rok7B7 also controls the production of antibiotics such as actinorhodin (ACT, 8), undecylprodigiosin (RED, 21), and calcium-dependent antibiotic (CDA, 22) in Streptomyces coelicolor M145 (Figure 3b). Chen et al. and Shu et al. reported that under stress associated with high concentrations of glutamate, AfsQ1/Q2 is important not only for maintaining the metabolic homeostasis of nutrient utilization but also for biosynthesis of antibiotics such as ACT, RED, CDA and the yellow pigment coelimycin P2 in Streptomyces coelicolor strains M145 [60,61].
Depletion of a metal component essential for growth activates the production of secondary metabolites with chelating activity, which enables the actinomycetes to acquire that metal (Figure 3c). Béchet and Blondeau reported that Streptomyces aureofaciens ATCC 10762 produces substantial amounts of tetracycline (23) only when the defined medium is deprived of iron [62]. Although not a compound obtained using approaches for activating silent genes, Wang et al. discovered SF2768, a metabolite produced by Streptomyces thioluteus that chelates copper as a chalkophore [63]. However, it has been suggested that activation of secondary metabolism in actinomycetes may depend on various metals. Indeed, it has been reported that adding to the culture medium rare metals not normally used as medium components can activate secondary metabolite biosynthetic genes. For example, Kamijo et al. found that Streptomyces sp. YB-1 produced reddish-purple pigment(s) only in the presence of a rare earth metal, ytterbium (Yb), but not in the presence of other metals. Structural analyses revealed that this pigment is a type of naphthoquinone similar to nanaomycin (24) [64]. Furthermore, Tanaka et al. showed that low concentrations of the rare earth elements scandium (Sc) and/or lanthanum (La) markedly activate (2.5 to 12-fold increase) the expression of nine secondary metabolite biosynthetic gene clusters in Streptomyces coelicolor A3(2) [65].
Furthermore, it has been reported that changing the pH of the culture medium can also result in the production of new substances (Figure 3d). Jiang et al. achieved a higher yield of validamycin A (25) from Streptomyces hygroscopicus 5008 together with more rapid protein synthesis and sugar consumption by subjecting cultures to one or more NaOH shocks [66]. Ren et al. obtained enhanced ε-poly-ʟ-lysine (ε-PL, 26) production by acidic pH shock of Streptomyces sp. M-Z18, achieving 52.5% higher production than controls without pH shock [67]. Conversely, the present authors were able to increase the production of antarlides, which have a 22-membered ring macrocyclic structure, by adding disodium hydrogen phosphate to the culture medium of Streptomyces sp. BB47 to prevent the pH of the culture medium from becoming too acidic [17]. In addition, Mehmood et al. reported that the oxygen supply controls the production of pristinamycins 27 in Streptomyces pristinaespiralis (Figure 3e) [68]. Takano et al. discovered the light-induced production of carotenoid pigments, including isorenieratene (28) and β-carotene (29), in Streptomyces coelicolor A3(2) (Figure 3f) [69]. Two transcriptional regulators, LitR and LitS, have been proposed as playing central roles in this light-induction mechanism in actinomycetes.
Thus, each element in the culture environment is important for the activation of silent genes. The authors’ group has been working on methods to activate these genes by increasing the culture temperature above the normal level.
Temperature – heat-shock metabolites (HSMs) – metabolites for which production is activated by high-temperature culture
Several examples of high-temperature culture of actinomycetes have been reported prior to the authors’ examples as studies attempting to increase the production of the targeted metabolites (Figure 4a). For example, Doull et al. achieved an increase in the production of jadomycin B (30) produced by Streptomyces venezuelae ISP5230 by heat shock at 42 °C for 1 h [70]. Similarly, James et al. increased the production of granaticin (31) by Streptomyces thermoviolaceus NCIB 10076 by changing the culture temperature [71]. They showed that the yield was best at 45 °C, whereas the rate of synthesis was highest at 37 °C. However, there have been no exhaustive analyses of high-temperature culture of actinomycetes. Thus, Saito et al. decided to test on a large scale whether high-temperature culture of actinomycetes can activate silent genes.
Figure 4: Structures of secondary metabolites obtained by high-temperature culture of actinomycetes.
Figure 4: Structures of secondary metabolites obtained by high-temperature culture of actinomycetes.
First, an in-house library of 3,160 strains of actinomycetes widely isolated from Japanese soil was searched to identify actinomycete strains capable of growing at high temperatures (45 °C). This screening identified 57 actinomycete strains capable of growing at 45 °C. Comparative analysis of metabolites produced by these 57 actinomycetes at room temperature or high temperature revealed that almost half of the strains produced metabolites selectively at 45 °C. As these results were obtained using one type of medium, a set number of incubation days, and one analytical condition using LCMS, it is possible that additional metabolites could be detected by examining a variety of conditions.
Sixteen of the abovementioned strains were randomly selected and cultured on five different media to obtain metabolic profile data according to culture temperature. This analysis resulted in the detection of newly isolated metabolites, metabolites exhibiting enhanced production, metabolites exhibiting diminished production, and metabolites in which production ceased. Plotting the newly identified metabolites according to retention time and molecular weight determined by LCMS, it was expected that most of the metabolites would tend to be relatively polar and of low molecular weight. The results suggested that high-temperature culture induces the production of silent secondary metabolites in certain actinomycetes, and Saito et al. therefore designated these compounds HSMs [72]. Then, the structures of these HSMs are attempted to elucidate (Figure 4b,c).
Gaudimycins A and B (32, 33) were identified as HSMs from Streptomyces sp. CA40. Palmu et al. discovered gaudimycins by heterologous expression of the biosynthetic gene clusters pga or cab, which were silent in the producing strain Streptomyces sp. PGA64 or Streptomyces sp. H021 relative to another host, Streptomyces lividans TK24 [73]. Resistomycin (34) [74], resistoflavin (35) [75], and tetracenomycin D (36) [76] were also identified as HSMs from Streptomyces sp. HN66. Carlson et al. reported the production of resistomycin by co-cultivating Streptomyces sp. B033 and Burkholderia vietnamiensis ATCC BAA-248 [77]. These results suggest that high-temperature culture of actinomycetes activates the production of silent secondary metabolites.
Further investigation of HSMs led to the discovery of three new compounds from different actinomycete strains. The first HSM is murecholamide (37), produced by Streptomyces sp. AY2 [72]. This substance has a skeleton in which a substructure of desferrioxamine (DFO, 38), a well-known secondary metabolite [78], is condensed at the end of the steroid skeleton. It is thought that DFO is produced as a result of the activation of a silent gene, whereas the steroid skeleton may be a component of the culture medium. At a concentration of 182 μM, murecholamide exhibits biological activity in inhibiting the migration of HT29 cancer cells. The second HSM is noaoxazole (39), which was discovered in Streptomyces sp. HR41 [79]. This substance has a chemical structure with a methylated oxazole ring at the end of a chain-like structure. The structure of noaoxazole is similar to that of inthomycin and oxazolomycin [80,81], but the methylated oxazole ring is a relatively valuable structure found elsewhere only in curromycins A and B (40, 41) and neocurromycin [82,83]. As with curromycin A, the end product of noaoxazole is expected to be different, but its discovery has not been reported to date. Noaoxazole has an activating effect on Notch signaling, which may result in inhibition of the malignant transformation of some cancers [84,85]. The third HSM is dihydromaniwamycin E (42), which was discovered in Streptomyces sp. JA74 [86]. Maniwamycin E (43) [87] was also discovered from this strain as a metabolite for which production is enhanced by high-temperature culture. These substances have an azoxy structure, which is relatively rare in the natural environment [88]. The stereochemistry of dihydromaniwamycin E was determined by total synthesis. The biological activities of these maniwamycins were also evaluated, and they showed antiviral activity against influenza (H1N1) virus and the causative agent of COVID-19, SARS-CoV-2. This was the first report of such activity for a natural product having an azoxy structure.
Saito et al. are now interested in determining how and why these HSMs are produced. First, when actinomycetes sense heat stress, repression mediated by heat shock sensor proteins such as HrcA, HspR, and RheA is released. The expression of various genes, such as those encoding heat shock proteins (HSPs), is then activated [89-91]. Lu et al. reported that the heat shock sensor HspR regulates the production of avermectin in Streptomyces avermitilis [92]. Further investigation is needed to determine if HSM production is induced after short periods of thermal stress and whether this production is regulated by such heat shock sensors. Although long-term high-temperature culture experiments are employed in this study, reactive oxygen species (ROS) may exert a stronger effect than high temperature. In actinomycetes, several sensor proteins for ROS have been reported (e.g., SoxR and OxyR), which upon sensing ROS, release their repression and activate the expression of various genes [93-95]. Wei et al. reported that the production of validamycin A (25) by Streptomyces hygroscopicus 5008 could be activated at the transcriptional level by simply adding hydrogen peroxide (H2O2; which generates ROS) to the culture medium [96]. In addition, there are also reports of cases in which denatured proteins are folded by molecular chaperones in high-temperature environments, and such activity may affect the production of secondary metabolites [97]. Saito et al. are currently attempting to determine how HSMs are produced.
Some studies have reported that secondary metabolites produced by actinomycetes are used physiologically by the producing strains and for communication with other strains. For example, Tenconi et al. reported that under certain conditions, the biosynthesis of undecylprodigiosin (21) is always triggered in the dying zone of the mycelial network of Streptomyces coelicolor M145 prior to morphological differentiation, right after an initial round of cell death [98]. They hypothesized that under certain circumstances, production of antiproliferative agents could play a role in the genetically programmed death of the producing organism. In addition, Nishiyama et al. suggested that actinorhodin (8) produced by Streptomyces coelicolor A3(2) functions as an organocatalyst to kill bacteria by catalyzing the production of toxic levels of H2O2 [99]. They also suggested that living organisms produce and use such metabolites as biocatalysts in nature. Therefore, it is thought that the production of HSMs has some physiological significance. For example, other microbial species reportedly activate energy metabolism and strengthen the cell membrane or walls in high-temperature environments as a means of ensuring thermotolerance [100-102]. As this suggests that HSMs may support thermotolerance, Saito et al. are also currently trying to determine why HSMs are produced.
Co-culture
It is also important to maintain the culture environment in a state close to that of the natural environment in order to activate silent genes in actinomycetes. Actinomycetes mainly inhabit soils, but they are widely symbiotic with plants [103], insects [104], and other organisms [105,106], and there are reports of their secondary metabolites controlling their own physiological functions or functioning as communication molecules [107,108]. Therefore, various studies have attempted to activate secondary metabolite biosynthetic genes under culture conditions that mimic the natural environment. In particular, the co-culture method, in which multiple microorganisms are cultured simultaneously, has been used in many studies examining silent gene activation (Figure 5a).
Figure 5: Structures of secondary metabolites obtained by co-culture of actinomycetes with other microorganisms or animal cells.
Figure 5: Structures of secondary metabolites obtained by co-culture of actinomycetes with other microorganis...
For example, Krespach et al. reported that Streptomyces iranensis secretes azalomycin F3a (44) in the presence of Aspergillus nidulans [109]. Interestingly, A. nidulans also reacted to the presence of azalomycin F3a by producing orsellinic acid, lecanoric acid, and the compounds F-9775A and F-9775B. Lee et al. discovered that secondary metabolism in Streptomyces coelicolor A3(2) M145 is activated by iron competition during co-culture with Myxococcus xanthus [110]. Co-culture promotes the production of the siderophore myxochelin in M. xanthus, which enhances the iron-scavenging capacity of M. xanthus and places S. coelicolor A3(2) M145 under iron starvation conditions. This chemical interaction activates the actinorhodin (8) biosynthetic gene cluster in S. coelicolor A3(2) M145. Onaka’s group reported that co-cultivation of Streptomyces and mycolic acid-containing bacteria (MACB) activates secondary metabolism [111]. In their method, direct attachment by live MACB cells is thought to alter secondary metabolism in the Streptomyces cells [112]. Using this method, Onaka et al. identified a variety of new compounds, such as catenulobactins A and B (45, 46) [113], dracolactams A and B (47, 48) [114], and chojalactones A–C (49–51) [115]. Finally, although not an example of co-culture, Chevrette et al. found that the potential secondary metabolic capacity differs between soil-derived and insect-derived actinomycetes [116]. Insect-associated Streptomyces inhibit antimicrobial-resistant pathogens to a greater degree than soil Streptomyces. This suggests that the evolutionary trajectories of Streptomyces from the insect microbiome influence their biosynthetic potential and ability to inhibit resistant pathogens. Against this background, a unique case of activation of secondary metabolite biosynthetic genes by co-culture of actinomycetes and animal cells has been reported, which we outline in detail below.
If microorganisms and animal cells are used, one might first imagine the interaction between humans and intestinal bacteria. Indeed, several examples of co-culture of human cells and intestinal bacteria have been reported, and the changes in gene expression on the host side during such co-culture experiments have been well studied [117,118]. On the other hand, unlike intestinal bacteria, pathogenic microorganisms that adversely affect human health also interact with host cells when they invade the body [119,120]. Noting this, Ishibashi and Arai et al., hypothesized that when pathogenic microorganisms invade the body, they are subjected to xenocidal stress from immune cells, which activates silent genes and produces defensive compounds as a countermeasure. Therefore, the pathogenic actinomycetes were co-cultured with J774.1 mouse macrophage-like cells (Figure 5b).
The first compound isolated was nocarjamide (52), a cyclic nonapeptide, discovered by co-culturing Nocardia tenerifensis IFM 10554T with J774.1 cells [121]. Nocarjamide exhibited biological activity by activating Wnt signaling at an effective concentration of 20–40 μM. Wnt signaling plays an important role in a number of vital processes, such as the formation of various tissues and the differentiation/proliferation of eukaryotic cells [122]. It is interesting that substances that affect Wnt signaling can be produced in response to the interaction between animal cells and microorganisms. In addition, dehydropropylpantothenamide (53) was also identified using the same co-culture combination [123]. The second group of compounds identified included uniformides A and B (54, 55), which were discovered by co-culturing Nocardia uniformis IFM0856T and J774.1 cells [124,125]. Uniformides were shown to suppress the production of nitric oxide, IL-6, and IL-1β by inhibiting the NF-κB pathway. Because NF-κB signaling plays a central role in the immune response [126], it is interesting that substances that regulate the pathway were produced by co-culture.
Thus, co-culture of animal cells and microorganisms may also be useful as a method for activating silent genes. However, it is important to determine what types of interactions between animal cells and microorganisms activate secondary metabolism in order to understand communication within the human body. For example, co-culture experiments using certain microorganisms have shown that physical contact between live microorganisms is important for the activation of secondary metabolism [112]. In addition, as mentioned above, various substances and changes in the concentrations of metal ions can affect secondary metabolism, and secondary metabolite production may thus be activated by these factors [110].
Conclusion
Natural products hold great potential as leads in drug development and as useful tools in chemical biology research. Therefore, attempts to obtain novel secondary metabolites by activating the latent metabolic potential of actinomycetes are gaining momentum. In this review, methods used to search for new secondary metabolites, focusing mainly on high-temperature culture of actinomycetes and the co-culture of microorganisms and animal cells, were outlined. The mechanisms by which these silent secondary metabolites are produced should be specific to each method. Elucidation of these mechanisms will directly lead to the development of new silent gene activation methods. In these approaches, there are some disadvantages associated with limited adaptability of microbial species (high-temperature culture) and somewhat complex experimental manipulations (co-culture method). It is important to overcome these disadvantages, broaden the range of adaptable microbial species, and develop a simple method exhibiting high activation efficiency.
The metabolites produced by the activation of silent genes are expected to be physiologically significant. In the case of high-temperature culture or co-culture methods, these compounds may play roles in thermotolerance or communication between different species, respectively. Analyses of the functionality of such metabolites may also lead to the elucidation of new life phenomena. However, in recent years, it has not only become increasingly difficult to obtain novel secondary metabolites from natural products, but applied research using natural products, such as drug discovery studies, has been losing momentum. In drug discovery studies in the field of chemical biology, it may be important to investigate the compounds obtained by silent gene activation. It is expected that compounds useful in a variety of fields will be discovered by making full use of such silent gene activation methods.
Acknowledgements
The graphical abstract was created by Science Graphics Co., Ltd. This content is not subject to CC BY 4.0.
Funding
This study was supported by Grants-in-Aid for Early Career Scientists (nos. 20K15461 and 23K13897 to S.S.); a Grant-in-Aid for Scientific Research (B) (no. 21H02639 to M.A.A.) from the Japan Society for the Promotion of Science (JSPS); and Grants-in-Aid for Transformative Research Area (A) “Latent Chemical Space” (nos. 23H04880 and 23H04884 to M.A.A.) from the Ministry of Education, Culture, Sports, Science and Technology, Japan. M.A.A. thanks the Mitsubishi Foundation. M.A.A. was the recipient of a JSBBA Innovative Research Program Award. S.S. was the recipient of a Noda Institute for Scientific Research (NISR) Young Investigator Research Grant.
Data Availability Statement
Data sharing is not applicable as no new data was generated or analyzed in this study.
References
-
Newman, D. J.; Cragg, G. M. J. Nat. Prod. 2020, 83, 770–803. doi:10.1021/acs.jnatprod.9b01285
Return to citation in text: [1] -
Demain, A. L.; Sanchez, S. J. Antibiot. 2009, 62, 5–16. doi:10.1038/ja.2008.16
Return to citation in text: [1] -
Gonzalez Ronquillo, M.; Angeles Hernandez, J. C. Food Control 2017, 72B, 255–267. doi:10.1016/j.foodcont.2016.03.001
Return to citation in text: [1] -
Zimbro, M. J.; Power, D. A.; Miller, S. M.; Wilson, G. E.; Johnson, J. A., Eds. DifcoTM & BBLTM Manual. Manual of Microbiological Culture Media, 2nd ed.; Becton Dickinson and Company: Sparks, MD, USA, 2009.
Return to citation in text: [1] -
Ryu, A. H.; Eckalbar, W. L.; Kreimer, A.; Yosef, N.; Ahituv, N. Sci. Rep. 2017, 7, 7533. doi:10.1038/s41598-017-07757-w
Return to citation in text: [1] -
Cell Culture Antibiotic Selection Guide. https://www.sigmaaldrich.com/life-science/cell-culture/learning-center/antibiotic-selector.html (accessed Dec 7, 2023).
Return to citation in text: [1] -
Villalobos-Delgado, L. H.; Nevárez-Moorillon, G. V.; Caro, I.; Quinto, E. J.; Mateo, J. Natural antimicrobial agents to improve foods shelf life. In Food Quality and Shelf Life; Galanakis, C. M., Ed.; Elsevier: Amsterdam, Netherlands, 2019; pp 125–157. doi:10.1016/b978-0-12-817190-5.00004-5
Return to citation in text: [1] -
Srianta, I.; Ristiarini, S.; Nugerahani, I.; Sen, S. K.; Zhang, B. B.; Xu, G. R.; Blanc, P. J. Int. Food Res. J. 2014, 21, 1–12.
Return to citation in text: [1] -
Purich, D. The Inhibitor Index: A Desk Reference on Enzyme Inhibitors, Receptor Antagonists, Drugs, Toxins, Poisons, Biologics, and Therapeutic Leads, 1st ed.; CRC Press: Boca Raton, FL, USA, 2017. doi:10.1201/9781315184289
Return to citation in text: [1] -
Tyc, O.; Song, C.; Dickschat, J. S.; Vos, M.; Garbeva, P. Trends Microbiol. 2017, 25, 280–292. doi:10.1016/j.tim.2016.12.002
Return to citation in text: [1] -
Schatz, A.; Bugle, E.; Waksman, S. A. Proc. Soc. Exp. Biol. Med. 1944, 55, 66–69. doi:10.3181/00379727-55-14461
Return to citation in text: [1] -
Burg, R. W.; Miller, B. M.; Baker, E. E.; Birnbaum, J.; Currie, S. A.; Hartman, R.; Kong, Y.-L.; Monaghan, R. L.; Olson, G.; Putter, I.; Tunac, J. B.; Wallick, H.; Stapley, E. O.; Oiwa, R.; Ōmura, S. Antimicrob. Agents Chemother. 1979, 15, 361–367. doi:10.1128/aac.15.3.361
Return to citation in text: [1] -
Watve, M. G.; Tickoo, R.; Jog, M. M.; Bhole, B. D. Arch. Microbiol. 2001, 176, 386–390. doi:10.1007/s002030100345
Return to citation in text: [1] -
Lacey, H. J.; Rutledge, P. J. Molecules 2022, 27, 887. doi:10.3390/molecules27030887
Return to citation in text: [1] -
Tashiro, E.; Imoto, M. Arch. Pharmacal Res. 2015, 38, 1651–1660. doi:10.1007/s12272-015-0633-4
Return to citation in text: [1] -
Kawamura, T.; Fujimaki, T.; Hamanaka, N.; Torii, K.; Kobayashi, H.; Takahashi, Y.; Igarashi, M.; Kinoshita, N.; Nishimura, Y.; Tashiro, E.; Imoto, M. J. Antibiot. 2010, 63, 601–605. doi:10.1038/ja.2010.98
Return to citation in text: [1] -
Saito, S.; Fujimaki, T.; Panbangred, W.; Igarashi, Y.; Imoto, M. Angew. Chem., Int. Ed. 2016, 55, 2728–2732. doi:10.1002/anie.201510079
Return to citation in text: [1] [2] -
Fujimaki, T.; Saito, S.; Imoto, M. J. Antibiot. 2017, 70, 328–330. doi:10.1038/ja.2016.162
Return to citation in text: [1] -
Saito, S.; Fujimaki, T.; Panbangred, W.; Sawa, R.; Igarashi, Y.; Imoto, M. J. Antibiot. 2017, 70, 595–600. doi:10.1038/ja.2017.6
Return to citation in text: [1] -
Tiwari, K.; Gupta, R. K. Crit. Rev. Biotechnol. 2012, 32, 108–132. doi:10.3109/07388551.2011.562482
Return to citation in text: [1] -
Saito, S.; Atsumi, K.; Zhou, T.; Fukaya, K.; Urabe, D.; Oku, N.; Karim, M. R. U.; Komaki, H.; Igarashi, Y. Beilstein J. Org. Chem. 2020, 16, 1100–1110. doi:10.3762/bjoc.16.97
Return to citation in text: [1] -
Saito, S.; Indo, K.; Oku, N.; Komaki, H.; Kawasaki, M.; Igarashi, Y. Beilstein J. Org. Chem. 2021, 17, 2939–2949. doi:10.3762/bjoc.17.203
Return to citation in text: [1] -
Saito, S.; Oku, N.; Igarashi, Y. J. Antibiot. 2022, 75, 44–47. doi:10.1038/s41429-021-00474-7
Return to citation in text: [1] -
Saito, S.; Xiaohanyao, Y.; Zhou, T.; Nakajima-Shimada, J.; Tashiro, E.; Triningsih, D. W.; Harunari, E.; Oku, N.; Igarashi, Y. J. Nat. Prod. 2022, 85, 1697–1703. doi:10.1021/acs.jnatprod.2c00137
Return to citation in text: [1] -
Igarashi, Y. J. Antibiot. 2023, 76, 365–383. doi:10.1038/s41429-023-00625-y
Return to citation in text: [1] -
Ohnishi, Y.; Ishikawa, J.; Hara, H.; Suzuki, H.; Ikenoya, M.; Ikeda, H.; Yamashita, A.; Hattori, M.; Horinouchi, S. J. Bacteriol. 2008, 190, 4050–4060. doi:10.1128/jb.00204-08
Return to citation in text: [1] -
Bentley, S. D.; Chater, K. F.; Cerdeño-Tárraga, A.-M.; Challis, G. L.; Thomson, N. R.; James, K. D.; Harris, D. E.; Quail, M. A.; Kieser, H.; Harper, D.; Bateman, A.; Brown, S.; Chandra, G.; Chen, C. W.; Collins, M.; Cronin, A.; Fraser, A.; Goble, A.; Hidalgo, J.; Hornsby, T.; Howarth, S.; Huang, C.-H.; Kieser, T.; Larke, L.; Murphy, L.; Oliver, K.; O'Neil, S.; Rabbinowitsch, E.; Rajandream, M.-A.; Rutherford, K.; Rutter, S.; Seeger, K.; Saunders, D.; Sharp, S.; Squares, R.; Squares, S.; Taylor, K.; Warren, T.; Wietzorrek, A.; Woodward, J.; Barrell, B. G.; Parkhill, J.; Hopwood, D. A. Nature 2002, 417, 141–147. doi:10.1038/417141a
Return to citation in text: [1] -
Ikeda, H.; Shin-ya, K.; Omura, S. J. Ind. Microbiol. Biotechnol. 2014, 41, 233–250. doi:10.1007/s10295-013-1327-x
Return to citation in text: [1] -
Liu, Y.; Zhou, H.; Shen, Q.; Dai, G.; Yan, F.; Li, X.; Ren, X.; Sun, Q.; Tang, Y.-J.; Zhang, Y.; Bian, X. Org. Lett. 2021, 23, 6967–6971. doi:10.1021/acs.orglett.1c02589
Return to citation in text: [1] -
Liu, S. H.; Wang, W.; Wang, K. B.; Zhang, B.; Li, W.; Shi, J.; Jiao, R. H.; Tan, R. X.; Ge, H. M. Org. Lett. 2019, 21, 3785–3788. doi:10.1021/acs.orglett.9b01237
Return to citation in text: [1] -
Khokhlov, A. S.; Tovarova, I. I.; Borisova, L. N.; Pliner, S. A.; Shevchenko, L. N.; Kornitskaia, E. Y.; Ivkina, N. S.; Rapoport, I. A. Dokl. Akad. Nauk SSSR 1967, 177, 232–235.
Return to citation in text: [1] -
Takano, E.; Nihira, T.; Hara, Y.; Jones, J. J.; Gershater, C. J. L.; Yamada, Y.; Bibb, M. J. Biol. Chem. 2000, 275, 11010–11016. doi:10.1074/jbc.275.15.11010
Return to citation in text: [1] -
Onaka, H.; Ando, N.; Nihira, T.; Yamada, Y.; Beppu, T.; Horinouchi, S. J. Bacteriol. 1995, 177, 6083–6092. doi:10.1128/jb.177.21.6083-6092.1995
Return to citation in text: [1] -
Takano, E.; Chakraburtty, R.; Nihira, T.; Yamada, Y.; Bibb, M. J. Mol. Microbiol. 2001, 41, 1015–1028. doi:10.1046/j.1365-2958.2001.02562.x
Return to citation in text: [1] -
HARA, O.; BEPPU, T. J. Antibiot. 1982, 35, 349–358. doi:10.7164/antibiotics.35.349
Return to citation in text: [1] -
Butler, M. J.; Takano, E.; Bruheim, P.; Jovetic, S.; Marinelli, F.; Bibb, M. J. Appl. Microbiol. Biotechnol. 2003, 61, 512–516. doi:10.1007/s00253-003-1277-8
Return to citation in text: [1] -
Kitani, S.; Miyamoto, K. T.; Takamatsu, S.; Herawati, E.; Iguchi, H.; Nishitomi, K.; Uchida, M.; Nagamitsu, T.; Omura, S.; Ikeda, H.; Nihira, T. Proc. Natl. Acad. Sci. U. S. A. 2011, 108, 16410–16415. doi:10.1073/pnas.1113908108
Return to citation in text: [1] -
Ohnishi, Y.; Furusho, Y.; Higashi, T.; Chun, H. K.; Furihata, K.; Sakuda, S.; Horinouchi, S. J. Antibiot. 2004, 57, 218–223. doi:10.7164/antibiotics.57.218
Return to citation in text: [1] -
Takano, E.; Kinoshita, H.; Mersinias, V.; Bucca, G.; Hotchkiss, G.; Nihira, T.; Smith, C. P.; Bibb, M.; Wohlleben, W.; Chater, K. Mol. Microbiol. 2005, 56, 465–479. doi:10.1111/j.1365-2958.2005.04543.x
Return to citation in text: [1] -
Sultan, S. P.; Kitani, S.; Miyamoto, K. T.; Iguchi, H.; Atago, T.; Ikeda, H.; Nihira, T. Appl. Microbiol. Biotechnol. 2016, 100, 9581–9591. doi:10.1007/s00253-016-7781-4
Return to citation in text: [1] -
Wilbanks, L. E.; Hennigan, H. E.; Martinez-Brokaw, C. D.; Lakkis, H.; Thormann, S.; Eggly, A. S.; Buechel, G.; Parkinson, E. I. ACS Chem. Biol. 2023, 18, 1624–1631. doi:10.1021/acschembio.3c00241
Return to citation in text: [1] -
Barajas, J. F.; Blake-Hedges, J. M.; Bailey, C. B.; Curran, S.; Keasling, J. D. Synth. Syst. Biotechnol. 2017, 2, 147–166. doi:10.1016/j.synbio.2017.08.005
Return to citation in text: [1] -
Du, D.; Katsuyama, Y.; Onaka, H.; Fujie, M.; Satoh, N.; Shin‐ya, K.; Ohnishi, Y. ChemBioChem 2016, 17, 1464–1471. doi:10.1002/cbic.201600167
Return to citation in text: [1] -
Du, D.; Katsuyama, Y.; Shin‐ya, K.; Ohnishi, Y. Angew. Chem., Int. Ed. 2018, 57, 1954–1957. doi:10.1002/anie.201709636
Return to citation in text: [1] -
Du, D.; Katsuyama, Y.; Horiuchi, M.; Fushinobu, S.; Chen, A.; Davis, T. D.; Burkart, M. D.; Ohnishi, Y. Nat. Chem. Biol. 2020, 16, 776–782. doi:10.1038/s41589-020-0530-0
Return to citation in text: [1] -
Seo, M.; Du, D.; Katsuyama, Y.; Katsuta, R.; Yajima, A.; Nukada, T.; Ohnishi, Y.; Ishigami, K. Biosci., Biotechnol., Biochem. 2021, 85, 148–153. doi:10.1093/bbb/zbaa023
Return to citation in text: [1] -
Ochi, K.; Okamoto, S.; Tozawa, Y.; Inaoka, T.; Hosaka, T.; Xu, J.; Kurosawa, K. Adv. Appl. Microbiol. 2004, 56, 155–184. doi:10.1016/s0065-2164(04)56005-7
Return to citation in text: [1] [2] -
Liu, L.; Li, S.; Sun, R.; Qin, X.; Ju, J.; Zhang, C.; Duan, Y.; Huang, Y. Org. Lett. 2020, 22, 4614–4619. doi:10.1021/acs.orglett.0c01224
Return to citation in text: [1] -
Seyedsayamdost, M. R. Proc. Natl. Acad. Sci. U. S. A. 2014, 111, 7266–7271. doi:10.1073/pnas.1400019111
Return to citation in text: [1] -
Han, E. J.; Lee, S. R.; Townsend, C. A.; Seyedsayamdost, M. R. ACS Chem. Biol. 2023, 18, 1854–1862. doi:10.1021/acschembio.3c00281
Return to citation in text: [1] -
Bode, H. B.; Bethe, B.; Höfs, R.; Zeeck, A. ChemBioChem 2002, 3, 619–627. doi:10.1002/1439-7633(20020703)3:7<619::aid-cbic619>3.0.co;2-9
Return to citation in text: [1] -
Schiewe, H.-J.; Zeeck, A. J. Antibiot. 1999, 52, 635–642. doi:10.7164/antibiotics.52.635
Return to citation in text: [1] -
Puder, C.; Krastel, P.; Zeeck, A. J. Nat. Prod. 2000, 63, 1258–1260. doi:10.1021/np0001373
Return to citation in text: [1] -
Puder, C.; Loya, S.; Hizi, A.; Zeeck, A. J. Nat. Prod. 2001, 64, 42–45. doi:10.1021/np000377i
Return to citation in text: [1] -
Rateb, M. E.; Houssen, W. E.; Harrison, W. T. A.; Deng, H.; Okoro, C. K.; Asenjo, J. A.; Andrews, B. A.; Bull, A. T.; Goodfellow, M.; Ebel, R.; Jaspars, M. J. Nat. Prod. 2011, 74, 1965–1971. doi:10.1021/np200470u
Return to citation in text: [1] -
Sproule, A.; Correa, H.; Decken, A.; Haltli, B.; Berrué, F.; Overy, D. P.; Kerr, R. G. Mar. Drugs 2019, 17, 347. doi:10.3390/md17060347
Return to citation in text: [1] -
Martín, J. F.; Liras, P. Front. Microbiol. 2020, 10, 3120. doi:10.3389/fmicb.2019.03120
Return to citation in text: [1] -
Swiatek, M. A.; Gubbens, J.; Bucca, G.; Song, E.; Yang, Y.-H.; Laing, E.; Kim, B.-G.; Smith, C. P.; van Wezel, G. P. J. Bacteriol. 2013, 195, 1236–1248. doi:10.1128/jb.02191-12
Return to citation in text: [1] -
Kim, S. H.; Traag, B. A.; Hasan, A. H.; McDowall, K. J.; Kim, B.-G.; van Wezel, G. P. Antonie van Leeuwenhoek 2015, 108, 201–213. doi:10.1007/s10482-015-0479-2
Return to citation in text: [1] -
Shu, D.; Chen, L.; Wang, W.; Yu, Z.; Ren, C.; Zhang, W.; Yang, S.; Lu, Y.; Jiang, W. Appl. Microbiol. Biotechnol. 2009, 81, 1149–1160. doi:10.1007/s00253-008-1738-1
Return to citation in text: [1] -
Chen, S.; Zheng, G.; Zhu, H.; He, H.; Chen, L.; Zhang, W.; Jiang, W.; Lu, Y. FEMS Microbiol. Lett. 2016, 363, fnw160. doi:10.1093/femsle/fnw160
Return to citation in text: [1] -
Béchet, M.; Blondeau, R. J. Appl. Microbiol. 1998, 84, 889–894. doi:10.1046/j.1365-2672.1998.00428.x
Return to citation in text: [1] -
Wang, L.; Zhu, M.; Zhang, Q.; Zhang, X.; Yang, P.; Liu, Z.; Deng, Y.; Zhu, Y.; Huang, X.; Han, L.; Li, S.; He, J. ACS Chem. Biol. 2017, 12, 3067–3075. doi:10.1021/acschembio.7b00897
Return to citation in text: [1] -
Kamijo, M.; Suzuki, T.; Kawai, K.; Fujii, T.; Murase, H. J. Biosci. Bioeng. 1999, 87, 340–343. doi:10.1016/s1389-1723(99)80042-5
Return to citation in text: [1] -
Tanaka, Y.; Hosaka, T.; Ochi, K. J. Antibiot. 2010, 63, 477–481. doi:10.1038/ja.2010.53
Return to citation in text: [1] -
Jiang, J.; Sun, Y.-F.; Tang, X.; He, C.-N.; Shao, Y.-L.; Tang, Y.-J.; Zhou, W.-W. Bioresour. Technol. 2018, 249, 234–240. doi:10.1016/j.biortech.2017.10.012
Return to citation in text: [1] -
Ren, X.-D.; Chen, X.-S.; Zeng, X.; Wang, L.; Tang, L.; Mao, Z.-G. Bioprocess Biosyst. Eng. 2015, 38, 1113–1125. doi:10.1007/s00449-015-1354-2
Return to citation in text: [1] -
Mehmood, N.; Olmos, E.; Goergen, J.-L.; Blanchard, F.; Ullisch, D.; Klöckner, W.; Büchs, J.; Delaunay, S. Biotechnol. Bioeng. 2011, 108, 2151–2161. doi:10.1002/bit.23177
Return to citation in text: [1] -
Takano, H.; Obitsu, S.; Beppu, T.; Ueda, K. J. Bacteriol. 2005, 187, 1825–1832. doi:10.1128/jb.187.5.1825-1832.2005
Return to citation in text: [1] -
Doull, J. L.; Ayer, S. W.; Singh, A. K.; Thibault, P. J. Antibiot. 1993, 46, 869–871. doi:10.7164/antibiotics.46.869
Return to citation in text: [1] -
James, P. D. A.; Edwards, C. J. Gen. Microbiol. 1989, 135, 1997–2003. doi:10.1099/00221287-135-7-1997
Return to citation in text: [1] -
Saito, S.; Kato, W.; Ikeda, H.; Katsuyama, Y.; Ohnishi, Y.; Imoto, M. J. Antibiot. 2020, 73, 203–210. doi:10.1038/s41429-020-0279-4
Return to citation in text: [1] [2] -
Palmu, K.; Ishida, K.; Mäntsälä, P.; Hertweck, C.; Metsä‐Ketelä, M. ChemBioChem 2007, 8, 1577–1584. doi:10.1002/cbic.200700140
Return to citation in text: [1] -
Brockmann, H.; Schmidt-Kastner, G. Naturwissenschaften 1951, 38, 479–480. doi:10.1007/bf00622090
Return to citation in text: [1] -
Eckardt, K.; Bradler, G.; Fritzsche, H.; Tresselt, D. Z. Chem. 1970, 10, 221. doi:10.1002/zfch.19700100607
Return to citation in text: [1] -
Yue, S.; Motamedi, H.; Wendt-Pienkowski, E.; Hutchinson, C. R. J. Bacteriol. 1986, 167, 581–586. doi:10.1128/jb.167.2.581-586.1986
Return to citation in text: [1] -
Carlson, S.; Tanouye, U.; Omarsdottir, S.; Murphy, B. T. J. Nat. Prod. 2015, 78, 381–387. doi:10.1021/np500767u
Return to citation in text: [1] -
Barona-Gómez, F.; Wong, U.; Giannakopulos, A. E.; Derrick, P. J.; Challis, G. L. J. Am. Chem. Soc. 2004, 126, 16282–16283. doi:10.1021/ja045774k
Return to citation in text: [1] -
Saito, S.; Suzuki, S.; Arai, M. A. J. Antibiot. 2022, 75, 509–513. doi:10.1038/s41429-022-00551-5
Return to citation in text: [1] -
Henkel, T.; Zeeck, A. Liebigs Ann. Chem. 1991, 367–373. doi:10.1002/jlac.199119910164
Return to citation in text: [1] -
Mori, T.; Takahashi, K.; Kashiwabara, M.; Uemura, D.; Katayama, C.; Iwadare, S.; Shizuri, Y.; Mitomo, R.; Nakano, F.; Matsuzaki, A. Tetrahedron Lett. 1985, 26, 1073–1076. doi:10.1016/s0040-4039(00)98515-0
Return to citation in text: [1] -
Ogura, M.; Nakayama, H.; Furihata, K.; Shimazu, A.; Seto, H.; Otake, N. J. Antibiot. 1985, 38, 669–673. doi:10.7164/antibiotics.38.669
Return to citation in text: [1] -
Hayakawa, Y.; Yaguchi, R.; Akimoto, M.; Kimata, S.; Shin-ya, K. J. Antibiot. 2020, 73, 790–793. doi:10.1038/s41429-020-0339-9
Return to citation in text: [1] -
Rampias, T.; Vgenopoulou, P.; Avgeris, M.; Polyzos, A.; Stravodimos, K.; Valavanis, C.; Scorilas, A.; Klinakis, A. Nat. Med. 2014, 20, 1199–1205. doi:10.1038/nm.3678
Return to citation in text: [1] -
Loganathan, S. K.; Schleicher, K.; Malik, A.; Quevedo, R.; Langille, E.; Teng, K.; Oh, R. H.; Rathod, B.; Tsai, R.; Samavarchi-Tehrani, P.; Pugh, T. J.; Gingras, A.-C.; Schramek, D. Science 2020, 367, 1264–1269. doi:10.1126/science.aax0902
Return to citation in text: [1] -
Saito, S.; Funayama, K.; Kato, W.; Okuda, M.; Kawamoto, M.; Matsubara, T.; Sato, T.; Sato, A.; Otsuguro, S.; Sasaki, M.; Orba, Y.; Sawa, H.; Maenaka, K.; Shindo, K.; Imoto, M.; Arai, M. A. J. Nat. Prod. 2022, 85, 2583–2591. doi:10.1021/acs.jnatprod.2c00550
Return to citation in text: [1] -
Fukumoto, A.; Murakami, C.; Anzai, Y.; Kato, F. J. Antibiot. 2016, 69, 395–399. doi:10.1038/ja.2015.126
Return to citation in text: [1] -
Wibowo, M.; Ding, L. J. Nat. Prod. 2020, 83, 3482–3491. doi:10.1021/acs.jnatprod.0c00725
Return to citation in text: [1] -
Grandvalet, C.; Servant, P.; Mazodier, P. Mol. Microbiol. 1997, 23, 77–84. doi:10.1046/j.1365-2958.1997.1811563.x
Return to citation in text: [1] -
Grandvalet, C.; Rapoport, G.; Mazodier, P. J. Bacteriol. 1998, 180, 5129–5134. doi:10.1128/jb.180.19.5129-5134.1998
Return to citation in text: [1] -
Servant, P.; Rapoport, G.; Mazodier, P. Microbiology (London, U. K.) 1999, 145, 2385–2391. doi:10.1099/00221287-145-9-2385
Return to citation in text: [1] -
Lu, X.; Wang, Q.; Yang, M.; Chen, Z.; Li, J.; Wen, Y. Appl. Environ. Microbiol. 2021, 87, e00473-21. doi:10.1128/aem.00473-21
Return to citation in text: [1] -
Hahn, J.-S.; Oh, S.-Y.; Chater, K. F.; Cho, Y.-H.; Roe, J.-H. J. Biol. Chem. 2000, 275, 38254–38260. doi:10.1074/jbc.m006079200
Return to citation in text: [1] -
Hahn, J.-S.; Oh, S.-Y.; Roe, J.-H. J. Bacteriol. 2002, 184, 5214–5222. doi:10.1128/jb.184.19.5214-5222.2002
Return to citation in text: [1] -
Dela Cruz, R.; Gao, Y.; Penumetcha, S.; Sheplock, R.; Weng, K.; Chander, M. J. Bacteriol. 2010, 192, 6428–6438. doi:10.1128/jb.00916-10
Return to citation in text: [1] -
Wei, Z.-H.; Bai, L.; Deng, Z.; Zhong, J.-J. Bioresour. Technol. 2011, 102, 1783–1787. doi:10.1016/j.biortech.2010.08.114
Return to citation in text: [1] -
Kudo, H.; Arakawa, G.-y.; Shirai, S.; Ogawa, M.; Shindo, H.; Hosaka, M.; Tokuoka, M. J. Biosci. Bioeng. 2022, 133, 235–242. doi:10.1016/j.jbiosc.2021.12.001
Return to citation in text: [1] -
Tenconi, E.; Traxler, M. F.; Hoebreck, C.; van Wezel, G. P.; Rigali, S. Front. Microbiol. 2018, 9, 1742. doi:10.3389/fmicb.2018.01742
Return to citation in text: [1] -
Nishiyama, T.; Hashimoto, Y.; Kusakabe, H.; Kumano, T.; Kobayashi, M. Proc. Natl. Acad. Sci. U. S. A. 2014, 111, 17152–17157. doi:10.1073/pnas.1417941111
Return to citation in text: [1] -
Sun, Q.-l.; Sun, Y.-y.; Zhang, J.; Luan, Z.-d.; Lian, C.; Liu, S.-q.; Yu, C. J. Proteomics 2019, 203, 103380. doi:10.1016/j.jprot.2019.103380
Return to citation in text: [1] -
Péter, M.; Gudmann, P.; Kóta, Z.; Török, Z.; Vígh, L.; Glatz, A.; Balogh, G. Int. J. Mol. Sci. 2021, 22, 13272. doi:10.3390/ijms222413272
Return to citation in text: [1] -
Wang, L.; Li, A.; Fang, J.; Wang, Y.; Chen, L.; Qiao, L.; Wang, W. Int. J. Mol. Sci. 2023, 24, 11822. doi:10.3390/ijms241411822
Return to citation in text: [1] -
Ayswaria, R.; Vasu, V.; Krishna, R. Crit. Rev. Microbiol. 2020, 46, 750–758. doi:10.1080/1040841x.2020.1828816
Return to citation in text: [1] -
Van Moll, L.; De Smet, J.; Cos, P.; Van Campenhout, L. Crit. Rev. Microbiol. 2021, 47, 562–579. doi:10.1080/1040841x.2021.1907302
Return to citation in text: [1] -
Sarmiento-Vizcaíno, A.; García, L. A.; Blanco, G. Arch. Microbiol. 2023, 205, 81. doi:10.1007/s00203-023-03422-1
Return to citation in text: [1] -
Chen, J.; Xu, L.; Zhou, Y.; Han, B. Mar. Drugs 2021, 19, 629. doi:10.3390/md19110629
Return to citation in text: [1] -
Takano, H.; Nishiyama, T.; Amano, S.-i.; Beppu, T.; Kobayashi, M.; Ueda, K. J. Ind. Microbiol. Biotechnol. 2016, 43, 143–148. doi:10.1007/s10295-015-1680-z
Return to citation in text: [1] -
Salwan, R.; Sharma, V. Microbiol. Res. 2020, 231, 126374. doi:10.1016/j.micres.2019.126374
Return to citation in text: [1] -
Krespach, M. K. C.; Stroe, M. C.; Netzker, T.; Rosin, M.; Zehner, L. M.; Komor, A. J.; Beilmann, J. M.; Krüger, T.; Scherlach, K.; Kniemeyer, O.; Schroeckh, V.; Hertweck, C.; Brakhage, A. A. Nat. Microbiol. 2023, 8, 1348–1361. doi:10.1038/s41564-023-01382-2
Return to citation in text: [1] -
Lee, N.; Kim, W.; Chung, J.; Lee, Y.; Cho, S.; Jang, K.-S.; Kim, S. C.; Palsson, B.; Cho, B.-K. ISME J. 2020, 14, 1111–1124. doi:10.1038/s41396-020-0594-6
Return to citation in text: [1] [2] -
Onaka, H.; Mori, Y.; Igarashi, Y.; Furumai, T. Appl. Environ. Microbiol. 2011, 77, 400–406. doi:10.1128/aem.01337-10
Return to citation in text: [1] -
Asamizu, S.; Ozaki, T.; Teramoto, K.; Satoh, K.; Onaka, H. PLoS One 2015, 10, e0142372. doi:10.1371/journal.pone.0142372
Return to citation in text: [1] [2] -
Hoshino, S.; Ozeki, M.; Awakawa, T.; Morita, H.; Onaka, H.; Abe, I. J. Nat. Prod. 2018, 81, 2106–2110. doi:10.1021/acs.jnatprod.8b00261
Return to citation in text: [1] -
Hoshino, S.; Okada, M.; Awakawa, T.; Asamizu, S.; Onaka, H.; Abe, I. Org. Lett. 2017, 19, 4992–4995. doi:10.1021/acs.orglett.7b02508
Return to citation in text: [1] -
Hoshino, S.; Wakimoto, T.; Onaka, H.; Abe, I. Org. Lett. 2015, 17, 1501–1504. doi:10.1021/acs.orglett.5b00385
Return to citation in text: [1] -
Chevrette, M. G.; Carlson, C. M.; Ortega, H. E.; Thomas, C.; Ananiev, G. E.; Barns, K. J.; Book, A. J.; Cagnazzo, J.; Carlos, C.; Flanigan, W.; Grubbs, K. J.; Horn, H. A.; Hoffmann, F. M.; Klassen, J. L.; Knack, J. J.; Lewin, G. R.; McDonald, B. R.; Muller, L.; Melo, W. G. P.; Pinto-Tomás, A. A.; Schmitz, A.; Wendt-Pienkowski, E.; Wildman, S.; Zhao, M.; Zhang, F.; Bugni, T. S.; Andes, D. R.; Pupo, M. T.; Currie, C. R. Nat. Commun. 2019, 10, 516. doi:10.1038/s41467-019-08438-0
Return to citation in text: [1] -
Zhang, J.; Hernandez-Gordillo, V.; Trapecar, M.; Wright, C.; Taketani, M.; Schneider, K.; Chen, W. L. K.; Stas, E.; Breault, D. T.; Carrier, R. L.; Voigt, C. A.; Griffith, L. G. Nat. Protoc. 2021, 16, 3874–3900. doi:10.1038/s41596-021-00562-w
Return to citation in text: [1] -
Nie, J.; Liao, W.; Zhang, Z.; Zhang, M.; Wen, Y.; Capanoglu, E.; Sarker, M. M. R.; Zhu, R.; Zhao, C. Curr. Res. Food Sci. 2023, 6, 100402. doi:10.1016/j.crfs.2022.11.021
Return to citation in text: [1] -
Toyotome, T.; Saito, S.; Koshizaki, Y.; Komatsu, R.; Matsuzawa, T.; Yaguchi, T. J. Infect. Chemother. 2020, 26, 321–323. doi:10.1016/j.jiac.2019.09.002
Return to citation in text: [1] -
Hamada, M.; Enomoto, N.; Yamashita, T.; Shimojima, M.; Tanno, D.; Ohana, N.; Toyokawa, M.; Takahashi, H.; Yaguchi, T. Int. J. Syst. Evol. Microbiol. 2023, 73, 005935. doi:10.1099/ijsem.0.005935
Return to citation in text: [1] -
Hara, Y.; Arai, M. A.; Toume, K.; Masu, H.; Sato, T.; Komatsu, K.; Yaguchi, T.; Ishibashi, M. Org. Lett. 2018, 20, 5831–5834. doi:10.1021/acs.orglett.8b02522
Return to citation in text: [1] -
Cadigan, K. M.; Liu, Y. I. J. Cell Sci. 2006, 119, 395–402. doi:10.1242/jcs.02826
Return to citation in text: [1] -
Hara, Y.; Arai, M. A.; Sakai, K.; Ishikawa, N.; Gonoi, T.; Yaguchi, T.; Ishibashi, M. J. Nat. Med. 2018, 72, 280–289. doi:10.1007/s11418-017-1161-y
Return to citation in text: [1] -
Hara, Y.; Watanabe, K.; Takaya, A.; Manome, T.; Yaguchi, T.; Ishibashi, M. Org. Lett. 2022, 24, 4998–5002. doi:10.1021/acs.orglett.2c02092
Return to citation in text: [1] -
Hara, Y.; Watanabe, K.; Takaya, A.; Ebihara, I.; Manome, T.; Arai, M. A.; Yaguchi, T.; Ishibashi, M. Org. Lett. 2022, 24, 5867. doi:10.1021/acs.orglett.2c02441
Return to citation in text: [1] -
Mussbacher, M.; Derler, M.; Basílio, J.; Schmid, J. A. Front. Immunol. 2023, 14, 1134661. doi:10.3389/fimmu.2023.1134661
Return to citation in text: [1]
47. | Ochi, K.; Okamoto, S.; Tozawa, Y.; Inaoka, T.; Hosaka, T.; Xu, J.; Kurosawa, K. Adv. Appl. Microbiol. 2004, 56, 155–184. doi:10.1016/s0065-2164(04)56005-7 |
48. | Liu, L.; Li, S.; Sun, R.; Qin, X.; Ju, J.; Zhang, C.; Duan, Y.; Huang, Y. Org. Lett. 2020, 22, 4614–4619. doi:10.1021/acs.orglett.0c01224 |
104. | Van Moll, L.; De Smet, J.; Cos, P.; Van Campenhout, L. Crit. Rev. Microbiol. 2021, 47, 562–579. doi:10.1080/1040841x.2021.1907302 |
49. | Seyedsayamdost, M. R. Proc. Natl. Acad. Sci. U. S. A. 2014, 111, 7266–7271. doi:10.1073/pnas.1400019111 |
105. | Sarmiento-Vizcaíno, A.; García, L. A.; Blanco, G. Arch. Microbiol. 2023, 205, 81. doi:10.1007/s00203-023-03422-1 |
106. | Chen, J.; Xu, L.; Zhou, Y.; Han, B. Mar. Drugs 2021, 19, 629. doi:10.3390/md19110629 |
100. | Sun, Q.-l.; Sun, Y.-y.; Zhang, J.; Luan, Z.-d.; Lian, C.; Liu, S.-q.; Yu, C. J. Proteomics 2019, 203, 103380. doi:10.1016/j.jprot.2019.103380 |
101. | Péter, M.; Gudmann, P.; Kóta, Z.; Török, Z.; Vígh, L.; Glatz, A.; Balogh, G. Int. J. Mol. Sci. 2021, 22, 13272. doi:10.3390/ijms222413272 |
102. | Wang, L.; Li, A.; Fang, J.; Wang, Y.; Chen, L.; Qiao, L.; Wang, W. Int. J. Mol. Sci. 2023, 24, 11822. doi:10.3390/ijms241411822 |
103. | Ayswaria, R.; Vasu, V.; Krishna, R. Crit. Rev. Microbiol. 2020, 46, 750–758. doi:10.1080/1040841x.2020.1828816 |
99. | Nishiyama, T.; Hashimoto, Y.; Kusakabe, H.; Kumano, T.; Kobayashi, M. Proc. Natl. Acad. Sci. U. S. A. 2014, 111, 17152–17157. doi:10.1073/pnas.1417941111 |
60. | Shu, D.; Chen, L.; Wang, W.; Yu, Z.; Ren, C.; Zhang, W.; Yang, S.; Lu, Y.; Jiang, W. Appl. Microbiol. Biotechnol. 2009, 81, 1149–1160. doi:10.1007/s00253-008-1738-1 |
61. | Chen, S.; Zheng, G.; Zhu, H.; He, H.; Chen, L.; Zhang, W.; Jiang, W.; Lu, Y. FEMS Microbiol. Lett. 2016, 363, fnw160. doi:10.1093/femsle/fnw160 |
62. | Béchet, M.; Blondeau, R. J. Appl. Microbiol. 1998, 84, 889–894. doi:10.1046/j.1365-2672.1998.00428.x |
57. | Martín, J. F.; Liras, P. Front. Microbiol. 2020, 10, 3120. doi:10.3389/fmicb.2019.03120 |
112. | Asamizu, S.; Ozaki, T.; Teramoto, K.; Satoh, K.; Onaka, H. PLoS One 2015, 10, e0142372. doi:10.1371/journal.pone.0142372 |
58. | Swiatek, M. A.; Gubbens, J.; Bucca, G.; Song, E.; Yang, Y.-H.; Laing, E.; Kim, B.-G.; Smith, C. P.; van Wezel, G. P. J. Bacteriol. 2013, 195, 1236–1248. doi:10.1128/jb.02191-12 |
59. | Kim, S. H.; Traag, B. A.; Hasan, A. H.; McDowall, K. J.; Kim, B.-G.; van Wezel, G. P. Antonie van Leeuwenhoek 2015, 108, 201–213. doi:10.1007/s10482-015-0479-2 |
52. | Schiewe, H.-J.; Zeeck, A. J. Antibiot. 1999, 52, 635–642. doi:10.7164/antibiotics.52.635 |
53. | Puder, C.; Krastel, P.; Zeeck, A. J. Nat. Prod. 2000, 63, 1258–1260. doi:10.1021/np0001373 |
54. | Puder, C.; Loya, S.; Hizi, A.; Zeeck, A. J. Nat. Prod. 2001, 64, 42–45. doi:10.1021/np000377i |
110. | Lee, N.; Kim, W.; Chung, J.; Lee, Y.; Cho, S.; Jang, K.-S.; Kim, S. C.; Palsson, B.; Cho, B.-K. ISME J. 2020, 14, 1111–1124. doi:10.1038/s41396-020-0594-6 |
55. | Rateb, M. E.; Houssen, W. E.; Harrison, W. T. A.; Deng, H.; Okoro, C. K.; Asenjo, J. A.; Andrews, B. A.; Bull, A. T.; Goodfellow, M.; Ebel, R.; Jaspars, M. J. Nat. Prod. 2011, 74, 1965–1971. doi:10.1021/np200470u |
56. | Sproule, A.; Correa, H.; Decken, A.; Haltli, B.; Berrué, F.; Overy, D. P.; Kerr, R. G. Mar. Drugs 2019, 17, 347. doi:10.3390/md17060347 |
111. | Onaka, H.; Mori, Y.; Igarashi, Y.; Furumai, T. Appl. Environ. Microbiol. 2011, 77, 400–406. doi:10.1128/aem.01337-10 |
50. | Han, E. J.; Lee, S. R.; Townsend, C. A.; Seyedsayamdost, M. R. ACS Chem. Biol. 2023, 18, 1854–1862. doi:10.1021/acschembio.3c00281 |
107. | Takano, H.; Nishiyama, T.; Amano, S.-i.; Beppu, T.; Kobayashi, M.; Ueda, K. J. Ind. Microbiol. Biotechnol. 2016, 43, 143–148. doi:10.1007/s10295-015-1680-z |
108. | Salwan, R.; Sharma, V. Microbiol. Res. 2020, 231, 126374. doi:10.1016/j.micres.2019.126374 |
51. | Bode, H. B.; Bethe, B.; Höfs, R.; Zeeck, A. ChemBioChem 2002, 3, 619–627. doi:10.1002/1439-7633(20020703)3:7<619::aid-cbic619>3.0.co;2-9 |
109. | Krespach, M. K. C.; Stroe, M. C.; Netzker, T.; Rosin, M.; Zehner, L. M.; Komor, A. J.; Beilmann, J. M.; Krüger, T.; Scherlach, K.; Kniemeyer, O.; Schroeckh, V.; Hertweck, C.; Brakhage, A. A. Nat. Microbiol. 2023, 8, 1348–1361. doi:10.1038/s41564-023-01382-2 |
63. | Wang, L.; Zhu, M.; Zhang, Q.; Zhang, X.; Yang, P.; Liu, Z.; Deng, Y.; Zhu, Y.; Huang, X.; Han, L.; Li, S.; He, J. ACS Chem. Biol. 2017, 12, 3067–3075. doi:10.1021/acschembio.7b00897 |
64. | Kamijo, M.; Suzuki, T.; Kawai, K.; Fujii, T.; Murase, H. J. Biosci. Bioeng. 1999, 87, 340–343. doi:10.1016/s1389-1723(99)80042-5 |
117. | Zhang, J.; Hernandez-Gordillo, V.; Trapecar, M.; Wright, C.; Taketani, M.; Schneider, K.; Chen, W. L. K.; Stas, E.; Breault, D. T.; Carrier, R. L.; Voigt, C. A.; Griffith, L. G. Nat. Protoc. 2021, 16, 3874–3900. doi:10.1038/s41596-021-00562-w |
118. | Nie, J.; Liao, W.; Zhang, Z.; Zhang, M.; Wen, Y.; Capanoglu, E.; Sarker, M. M. R.; Zhu, R.; Zhao, C. Curr. Res. Food Sci. 2023, 6, 100402. doi:10.1016/j.crfs.2022.11.021 |
65. | Tanaka, Y.; Hosaka, T.; Ochi, K. J. Antibiot. 2010, 63, 477–481. doi:10.1038/ja.2010.53 |
119. | Toyotome, T.; Saito, S.; Koshizaki, Y.; Komatsu, R.; Matsuzawa, T.; Yaguchi, T. J. Infect. Chemother. 2020, 26, 321–323. doi:10.1016/j.jiac.2019.09.002 |
120. | Hamada, M.; Enomoto, N.; Yamashita, T.; Shimojima, M.; Tanno, D.; Ohana, N.; Toyokawa, M.; Takahashi, H.; Yaguchi, T. Int. J. Syst. Evol. Microbiol. 2023, 73, 005935. doi:10.1099/ijsem.0.005935 |
115. | Hoshino, S.; Wakimoto, T.; Onaka, H.; Abe, I. Org. Lett. 2015, 17, 1501–1504. doi:10.1021/acs.orglett.5b00385 |
116. | Chevrette, M. G.; Carlson, C. M.; Ortega, H. E.; Thomas, C.; Ananiev, G. E.; Barns, K. J.; Book, A. J.; Cagnazzo, J.; Carlos, C.; Flanigan, W.; Grubbs, K. J.; Horn, H. A.; Hoffmann, F. M.; Klassen, J. L.; Knack, J. J.; Lewin, G. R.; McDonald, B. R.; Muller, L.; Melo, W. G. P.; Pinto-Tomás, A. A.; Schmitz, A.; Wendt-Pienkowski, E.; Wildman, S.; Zhao, M.; Zhang, F.; Bugni, T. S.; Andes, D. R.; Pupo, M. T.; Currie, C. R. Nat. Commun. 2019, 10, 516. doi:10.1038/s41467-019-08438-0 |
113. | Hoshino, S.; Ozeki, M.; Awakawa, T.; Morita, H.; Onaka, H.; Abe, I. J. Nat. Prod. 2018, 81, 2106–2110. doi:10.1021/acs.jnatprod.8b00261 |
114. | Hoshino, S.; Okada, M.; Awakawa, T.; Asamizu, S.; Onaka, H.; Abe, I. Org. Lett. 2017, 19, 4992–4995. doi:10.1021/acs.orglett.7b02508 |
71. | James, P. D. A.; Edwards, C. J. Gen. Microbiol. 1989, 135, 1997–2003. doi:10.1099/00221287-135-7-1997 |
72. | Saito, S.; Kato, W.; Ikeda, H.; Katsuyama, Y.; Ohnishi, Y.; Imoto, M. J. Antibiot. 2020, 73, 203–210. doi:10.1038/s41429-020-0279-4 |
69. | Takano, H.; Obitsu, S.; Beppu, T.; Ueda, K. J. Bacteriol. 2005, 187, 1825–1832. doi:10.1128/jb.187.5.1825-1832.2005 |
70. | Doull, J. L.; Ayer, S. W.; Singh, A. K.; Thibault, P. J. Antibiot. 1993, 46, 869–871. doi:10.7164/antibiotics.46.869 |
17. | Saito, S.; Fujimaki, T.; Panbangred, W.; Igarashi, Y.; Imoto, M. Angew. Chem., Int. Ed. 2016, 55, 2728–2732. doi:10.1002/anie.201510079 |
123. | Hara, Y.; Arai, M. A.; Sakai, K.; Ishikawa, N.; Gonoi, T.; Yaguchi, T.; Ishibashi, M. J. Nat. Med. 2018, 72, 280–289. doi:10.1007/s11418-017-1161-y |
68. | Mehmood, N.; Olmos, E.; Goergen, J.-L.; Blanchard, F.; Ullisch, D.; Klöckner, W.; Büchs, J.; Delaunay, S. Biotechnol. Bioeng. 2011, 108, 2151–2161. doi:10.1002/bit.23177 |
124. | Hara, Y.; Watanabe, K.; Takaya, A.; Manome, T.; Yaguchi, T.; Ishibashi, M. Org. Lett. 2022, 24, 4998–5002. doi:10.1021/acs.orglett.2c02092 |
125. | Hara, Y.; Watanabe, K.; Takaya, A.; Ebihara, I.; Manome, T.; Arai, M. A.; Yaguchi, T.; Ishibashi, M. Org. Lett. 2022, 24, 5867. doi:10.1021/acs.orglett.2c02441 |
66. | Jiang, J.; Sun, Y.-F.; Tang, X.; He, C.-N.; Shao, Y.-L.; Tang, Y.-J.; Zhou, W.-W. Bioresour. Technol. 2018, 249, 234–240. doi:10.1016/j.biortech.2017.10.012 |
121. | Hara, Y.; Arai, M. A.; Toume, K.; Masu, H.; Sato, T.; Komatsu, K.; Yaguchi, T.; Ishibashi, M. Org. Lett. 2018, 20, 5831–5834. doi:10.1021/acs.orglett.8b02522 |
67. | Ren, X.-D.; Chen, X.-S.; Zeng, X.; Wang, L.; Tang, L.; Mao, Z.-G. Bioprocess Biosyst. Eng. 2015, 38, 1113–1125. doi:10.1007/s00449-015-1354-2 |
122. | Cadigan, K. M.; Liu, Y. I. J. Cell Sci. 2006, 119, 395–402. doi:10.1242/jcs.02826 |
74. | Brockmann, H.; Schmidt-Kastner, G. Naturwissenschaften 1951, 38, 479–480. doi:10.1007/bf00622090 |
75. | Eckardt, K.; Bradler, G.; Fritzsche, H.; Tresselt, D. Z. Chem. 1970, 10, 221. doi:10.1002/zfch.19700100607 |
73. | Palmu, K.; Ishida, K.; Mäntsälä, P.; Hertweck, C.; Metsä‐Ketelä, M. ChemBioChem 2007, 8, 1577–1584. doi:10.1002/cbic.200700140 |
1. | Newman, D. J.; Cragg, G. M. J. Nat. Prod. 2020, 83, 770–803. doi:10.1021/acs.jnatprod.9b01285 |
112. | Asamizu, S.; Ozaki, T.; Teramoto, K.; Satoh, K.; Onaka, H. PLoS One 2015, 10, e0142372. doi:10.1371/journal.pone.0142372 |
110. | Lee, N.; Kim, W.; Chung, J.; Lee, Y.; Cho, S.; Jang, K.-S.; Kim, S. C.; Palsson, B.; Cho, B.-K. ISME J. 2020, 14, 1111–1124. doi:10.1038/s41396-020-0594-6 |
126. | Mussbacher, M.; Derler, M.; Basílio, J.; Schmid, J. A. Front. Immunol. 2023, 14, 1134661. doi:10.3389/fimmu.2023.1134661 |
7. | Villalobos-Delgado, L. H.; Nevárez-Moorillon, G. V.; Caro, I.; Quinto, E. J.; Mateo, J. Natural antimicrobial agents to improve foods shelf life. In Food Quality and Shelf Life; Galanakis, C. M., Ed.; Elsevier: Amsterdam, Netherlands, 2019; pp 125–157. doi:10.1016/b978-0-12-817190-5.00004-5 |
8. | Srianta, I.; Ristiarini, S.; Nugerahani, I.; Sen, S. K.; Zhang, B. B.; Xu, G. R.; Blanc, P. J. Int. Food Res. J. 2014, 21, 1–12. |
26. | Ohnishi, Y.; Ishikawa, J.; Hara, H.; Suzuki, H.; Ikenoya, M.; Ikeda, H.; Yamashita, A.; Hattori, M.; Horinouchi, S. J. Bacteriol. 2008, 190, 4050–4060. doi:10.1128/jb.00204-08 |
27. | Bentley, S. D.; Chater, K. F.; Cerdeño-Tárraga, A.-M.; Challis, G. L.; Thomson, N. R.; James, K. D.; Harris, D. E.; Quail, M. A.; Kieser, H.; Harper, D.; Bateman, A.; Brown, S.; Chandra, G.; Chen, C. W.; Collins, M.; Cronin, A.; Fraser, A.; Goble, A.; Hidalgo, J.; Hornsby, T.; Howarth, S.; Huang, C.-H.; Kieser, T.; Larke, L.; Murphy, L.; Oliver, K.; O'Neil, S.; Rabbinowitsch, E.; Rajandream, M.-A.; Rutherford, K.; Rutter, S.; Seeger, K.; Saunders, D.; Sharp, S.; Squares, R.; Squares, S.; Taylor, K.; Warren, T.; Wietzorrek, A.; Woodward, J.; Barrell, B. G.; Parkhill, J.; Hopwood, D. A. Nature 2002, 417, 141–147. doi:10.1038/417141a |
28. | Ikeda, H.; Shin-ya, K.; Omura, S. J. Ind. Microbiol. Biotechnol. 2014, 41, 233–250. doi:10.1007/s10295-013-1327-x |
82. | Ogura, M.; Nakayama, H.; Furihata, K.; Shimazu, A.; Seto, H.; Otake, N. J. Antibiot. 1985, 38, 669–673. doi:10.7164/antibiotics.38.669 |
83. | Hayakawa, Y.; Yaguchi, R.; Akimoto, M.; Kimata, S.; Shin-ya, K. J. Antibiot. 2020, 73, 790–793. doi:10.1038/s41429-020-0339-9 |
4. | Zimbro, M. J.; Power, D. A.; Miller, S. M.; Wilson, G. E.; Johnson, J. A., Eds. DifcoTM & BBLTM Manual. Manual of Microbiological Culture Media, 2nd ed.; Becton Dickinson and Company: Sparks, MD, USA, 2009. |
5. | Ryu, A. H.; Eckalbar, W. L.; Kreimer, A.; Yosef, N.; Ahituv, N. Sci. Rep. 2017, 7, 7533. doi:10.1038/s41598-017-07757-w |
6. | Cell Culture Antibiotic Selection Guide. https://www.sigmaaldrich.com/life-science/cell-culture/learning-center/antibiotic-selector.html (accessed Dec 7, 2023). |
29. | Liu, Y.; Zhou, H.; Shen, Q.; Dai, G.; Yan, F.; Li, X.; Ren, X.; Sun, Q.; Tang, Y.-J.; Zhang, Y.; Bian, X. Org. Lett. 2021, 23, 6967–6971. doi:10.1021/acs.orglett.1c02589 |
3. | Gonzalez Ronquillo, M.; Angeles Hernandez, J. C. Food Control 2017, 72B, 255–267. doi:10.1016/j.foodcont.2016.03.001 |
20. | Tiwari, K.; Gupta, R. K. Crit. Rev. Biotechnol. 2012, 32, 108–132. doi:10.3109/07388551.2011.562482 |
79. | Saito, S.; Suzuki, S.; Arai, M. A. J. Antibiot. 2022, 75, 509–513. doi:10.1038/s41429-022-00551-5 |
21. | Saito, S.; Atsumi, K.; Zhou, T.; Fukaya, K.; Urabe, D.; Oku, N.; Karim, M. R. U.; Komaki, H.; Igarashi, Y. Beilstein J. Org. Chem. 2020, 16, 1100–1110. doi:10.3762/bjoc.16.97 |
22. | Saito, S.; Indo, K.; Oku, N.; Komaki, H.; Kawasaki, M.; Igarashi, Y. Beilstein J. Org. Chem. 2021, 17, 2939–2949. doi:10.3762/bjoc.17.203 |
23. | Saito, S.; Oku, N.; Igarashi, Y. J. Antibiot. 2022, 75, 44–47. doi:10.1038/s41429-021-00474-7 |
24. | Saito, S.; Xiaohanyao, Y.; Zhou, T.; Nakajima-Shimada, J.; Tashiro, E.; Triningsih, D. W.; Harunari, E.; Oku, N.; Igarashi, Y. J. Nat. Prod. 2022, 85, 1697–1703. doi:10.1021/acs.jnatprod.2c00137 |
25. | Igarashi, Y. J. Antibiot. 2023, 76, 365–383. doi:10.1038/s41429-023-00625-y |
80. | Henkel, T.; Zeeck, A. Liebigs Ann. Chem. 1991, 367–373. doi:10.1002/jlac.199119910164 |
81. | Mori, T.; Takahashi, K.; Kashiwabara, M.; Uemura, D.; Katayama, C.; Iwadare, S.; Shizuri, Y.; Mitomo, R.; Nakano, F.; Matsuzaki, A. Tetrahedron Lett. 1985, 26, 1073–1076. doi:10.1016/s0040-4039(00)98515-0 |
12. | Burg, R. W.; Miller, B. M.; Baker, E. E.; Birnbaum, J.; Currie, S. A.; Hartman, R.; Kong, Y.-L.; Monaghan, R. L.; Olson, G.; Putter, I.; Tunac, J. B.; Wallick, H.; Stapley, E. O.; Oiwa, R.; Ōmura, S. Antimicrob. Agents Chemother. 1979, 15, 361–367. doi:10.1128/aac.15.3.361 |
15. | Tashiro, E.; Imoto, M. Arch. Pharmacal Res. 2015, 38, 1651–1660. doi:10.1007/s12272-015-0633-4 |
72. | Saito, S.; Kato, W.; Ikeda, H.; Katsuyama, Y.; Ohnishi, Y.; Imoto, M. J. Antibiot. 2020, 73, 203–210. doi:10.1038/s41429-020-0279-4 |
11. | Schatz, A.; Bugle, E.; Waksman, S. A. Proc. Soc. Exp. Biol. Med. 1944, 55, 66–69. doi:10.3181/00379727-55-14461 |
16. | Kawamura, T.; Fujimaki, T.; Hamanaka, N.; Torii, K.; Kobayashi, H.; Takahashi, Y.; Igarashi, M.; Kinoshita, N.; Nishimura, Y.; Tashiro, E.; Imoto, M. J. Antibiot. 2010, 63, 601–605. doi:10.1038/ja.2010.98 |
17. | Saito, S.; Fujimaki, T.; Panbangred, W.; Igarashi, Y.; Imoto, M. Angew. Chem., Int. Ed. 2016, 55, 2728–2732. doi:10.1002/anie.201510079 |
18. | Fujimaki, T.; Saito, S.; Imoto, M. J. Antibiot. 2017, 70, 328–330. doi:10.1038/ja.2016.162 |
19. | Saito, S.; Fujimaki, T.; Panbangred, W.; Sawa, R.; Igarashi, Y.; Imoto, M. J. Antibiot. 2017, 70, 595–600. doi:10.1038/ja.2017.6 |
78. | Barona-Gómez, F.; Wong, U.; Giannakopulos, A. E.; Derrick, P. J.; Challis, G. L. J. Am. Chem. Soc. 2004, 126, 16282–16283. doi:10.1021/ja045774k |
10. | Tyc, O.; Song, C.; Dickschat, J. S.; Vos, M.; Garbeva, P. Trends Microbiol. 2017, 25, 280–292. doi:10.1016/j.tim.2016.12.002 |
76. | Yue, S.; Motamedi, H.; Wendt-Pienkowski, E.; Hutchinson, C. R. J. Bacteriol. 1986, 167, 581–586. doi:10.1128/jb.167.2.581-586.1986 |
9. | Purich, D. The Inhibitor Index: A Desk Reference on Enzyme Inhibitors, Receptor Antagonists, Drugs, Toxins, Poisons, Biologics, and Therapeutic Leads, 1st ed.; CRC Press: Boca Raton, FL, USA, 2017. doi:10.1201/9781315184289 |
13. | Watve, M. G.; Tickoo, R.; Jog, M. M.; Bhole, B. D. Arch. Microbiol. 2001, 176, 386–390. doi:10.1007/s002030100345 |
14. | Lacey, H. J.; Rutledge, P. J. Molecules 2022, 27, 887. doi:10.3390/molecules27030887 |
77. | Carlson, S.; Tanouye, U.; Omarsdottir, S.; Murphy, B. T. J. Nat. Prod. 2015, 78, 381–387. doi:10.1021/np500767u |
32. | Takano, E.; Nihira, T.; Hara, Y.; Jones, J. J.; Gershater, C. J. L.; Yamada, Y.; Bibb, M. J. Biol. Chem. 2000, 275, 11010–11016. doi:10.1074/jbc.275.15.11010 |
30. | Liu, S. H.; Wang, W.; Wang, K. B.; Zhang, B.; Li, W.; Shi, J.; Jiao, R. H.; Tan, R. X.; Ge, H. M. Org. Lett. 2019, 21, 3785–3788. doi:10.1021/acs.orglett.9b01237 |
31. | Khokhlov, A. S.; Tovarova, I. I.; Borisova, L. N.; Pliner, S. A.; Shevchenko, L. N.; Kornitskaia, E. Y.; Ivkina, N. S.; Rapoport, I. A. Dokl. Akad. Nauk SSSR 1967, 177, 232–235. |
87. | Fukumoto, A.; Murakami, C.; Anzai, Y.; Kato, F. J. Antibiot. 2016, 69, 395–399. doi:10.1038/ja.2015.126 |
88. | Wibowo, M.; Ding, L. J. Nat. Prod. 2020, 83, 3482–3491. doi:10.1021/acs.jnatprod.0c00725 |
84. | Rampias, T.; Vgenopoulou, P.; Avgeris, M.; Polyzos, A.; Stravodimos, K.; Valavanis, C.; Scorilas, A.; Klinakis, A. Nat. Med. 2014, 20, 1199–1205. doi:10.1038/nm.3678 |
85. | Loganathan, S. K.; Schleicher, K.; Malik, A.; Quevedo, R.; Langille, E.; Teng, K.; Oh, R. H.; Rathod, B.; Tsai, R.; Samavarchi-Tehrani, P.; Pugh, T. J.; Gingras, A.-C.; Schramek, D. Science 2020, 367, 1264–1269. doi:10.1126/science.aax0902 |
86. | Saito, S.; Funayama, K.; Kato, W.; Okuda, M.; Kawamoto, M.; Matsubara, T.; Sato, T.; Sato, A.; Otsuguro, S.; Sasaki, M.; Orba, Y.; Sawa, H.; Maenaka, K.; Shindo, K.; Imoto, M.; Arai, M. A. J. Nat. Prod. 2022, 85, 2583–2591. doi:10.1021/acs.jnatprod.2c00550 |
46. | Seo, M.; Du, D.; Katsuyama, Y.; Katsuta, R.; Yajima, A.; Nukada, T.; Ohnishi, Y.; Ishigami, K. Biosci., Biotechnol., Biochem. 2021, 85, 148–153. doi:10.1093/bbb/zbaa023 |
47. | Ochi, K.; Okamoto, S.; Tozawa, Y.; Inaoka, T.; Hosaka, T.; Xu, J.; Kurosawa, K. Adv. Appl. Microbiol. 2004, 56, 155–184. doi:10.1016/s0065-2164(04)56005-7 |
42. | Barajas, J. F.; Blake-Hedges, J. M.; Bailey, C. B.; Curran, S.; Keasling, J. D. Synth. Syst. Biotechnol. 2017, 2, 147–166. doi:10.1016/j.synbio.2017.08.005 |
97. | Kudo, H.; Arakawa, G.-y.; Shirai, S.; Ogawa, M.; Shindo, H.; Hosaka, M.; Tokuoka, M. J. Biosci. Bioeng. 2022, 133, 235–242. doi:10.1016/j.jbiosc.2021.12.001 |
43. | Du, D.; Katsuyama, Y.; Onaka, H.; Fujie, M.; Satoh, N.; Shin‐ya, K.; Ohnishi, Y. ChemBioChem 2016, 17, 1464–1471. doi:10.1002/cbic.201600167 |
44. | Du, D.; Katsuyama, Y.; Shin‐ya, K.; Ohnishi, Y. Angew. Chem., Int. Ed. 2018, 57, 1954–1957. doi:10.1002/anie.201709636 |
45. | Du, D.; Katsuyama, Y.; Horiuchi, M.; Fushinobu, S.; Chen, A.; Davis, T. D.; Burkart, M. D.; Ohnishi, Y. Nat. Chem. Biol. 2020, 16, 776–782. doi:10.1038/s41589-020-0530-0 |
98. | Tenconi, E.; Traxler, M. F.; Hoebreck, C.; van Wezel, G. P.; Rigali, S. Front. Microbiol. 2018, 9, 1742. doi:10.3389/fmicb.2018.01742 |
38. | Ohnishi, Y.; Furusho, Y.; Higashi, T.; Chun, H. K.; Furihata, K.; Sakuda, S.; Horinouchi, S. J. Antibiot. 2004, 57, 218–223. doi:10.7164/antibiotics.57.218 |
39. | Takano, E.; Kinoshita, H.; Mersinias, V.; Bucca, G.; Hotchkiss, G.; Nihira, T.; Smith, C. P.; Bibb, M.; Wohlleben, W.; Chater, K. Mol. Microbiol. 2005, 56, 465–479. doi:10.1111/j.1365-2958.2005.04543.x |
40. | Sultan, S. P.; Kitani, S.; Miyamoto, K. T.; Iguchi, H.; Atago, T.; Ikeda, H.; Nihira, T. Appl. Microbiol. Biotechnol. 2016, 100, 9581–9591. doi:10.1007/s00253-016-7781-4 |
93. | Hahn, J.-S.; Oh, S.-Y.; Chater, K. F.; Cho, Y.-H.; Roe, J.-H. J. Biol. Chem. 2000, 275, 38254–38260. doi:10.1074/jbc.m006079200 |
94. | Hahn, J.-S.; Oh, S.-Y.; Roe, J.-H. J. Bacteriol. 2002, 184, 5214–5222. doi:10.1128/jb.184.19.5214-5222.2002 |
95. | Dela Cruz, R.; Gao, Y.; Penumetcha, S.; Sheplock, R.; Weng, K.; Chander, M. J. Bacteriol. 2010, 192, 6428–6438. doi:10.1128/jb.00916-10 |
41. | Wilbanks, L. E.; Hennigan, H. E.; Martinez-Brokaw, C. D.; Lakkis, H.; Thormann, S.; Eggly, A. S.; Buechel, G.; Parkinson, E. I. ACS Chem. Biol. 2023, 18, 1624–1631. doi:10.1021/acschembio.3c00241 |
96. | Wei, Z.-H.; Bai, L.; Deng, Z.; Zhong, J.-J. Bioresour. Technol. 2011, 102, 1783–1787. doi:10.1016/j.biortech.2010.08.114 |
33. | Onaka, H.; Ando, N.; Nihira, T.; Yamada, Y.; Beppu, T.; Horinouchi, S. J. Bacteriol. 1995, 177, 6083–6092. doi:10.1128/jb.177.21.6083-6092.1995 |
34. | Takano, E.; Chakraburtty, R.; Nihira, T.; Yamada, Y.; Bibb, M. J. Mol. Microbiol. 2001, 41, 1015–1028. doi:10.1046/j.1365-2958.2001.02562.x |
89. | Grandvalet, C.; Servant, P.; Mazodier, P. Mol. Microbiol. 1997, 23, 77–84. doi:10.1046/j.1365-2958.1997.1811563.x |
90. | Grandvalet, C.; Rapoport, G.; Mazodier, P. J. Bacteriol. 1998, 180, 5129–5134. doi:10.1128/jb.180.19.5129-5134.1998 |
91. | Servant, P.; Rapoport, G.; Mazodier, P. Microbiology (London, U. K.) 1999, 145, 2385–2391. doi:10.1099/00221287-145-9-2385 |
35. | HARA, O.; BEPPU, T. J. Antibiot. 1982, 35, 349–358. doi:10.7164/antibiotics.35.349 |
36. | Butler, M. J.; Takano, E.; Bruheim, P.; Jovetic, S.; Marinelli, F.; Bibb, M. J. Appl. Microbiol. Biotechnol. 2003, 61, 512–516. doi:10.1007/s00253-003-1277-8 |
37. | Kitani, S.; Miyamoto, K. T.; Takamatsu, S.; Herawati, E.; Iguchi, H.; Nishitomi, K.; Uchida, M.; Nagamitsu, T.; Omura, S.; Ikeda, H.; Nihira, T. Proc. Natl. Acad. Sci. U. S. A. 2011, 108, 16410–16415. doi:10.1073/pnas.1113908108 |
92. | Lu, X.; Wang, Q.; Yang, M.; Chen, Z.; Li, J.; Wen, Y. Appl. Environ. Microbiol. 2021, 87, e00473-21. doi:10.1128/aem.00473-21 |
© 2024 Saito and Arai; licensee Beilstein-Institut.
This is an open access article licensed under the terms of the Beilstein-Institut Open Access License Agreement (https://www.beilstein-journals.org/bjoc/terms), which is identical to the Creative Commons Attribution 4.0 International License (https://creativecommons.org/licenses/by/4.0). The reuse of material under this license requires that the author(s), source and license are credited. Third-party material in this article could be subject to other licenses (typically indicated in the credit line), and in this case, users are required to obtain permission from the license holder to reuse the material.