Abstract
We report novel pseudorotaxanes based on the complexation between pillar[4]arene[1]quinone and 1,10-dibromodecane. The complexation is found to have a 1:1 host–guest complexation stoichiometry in chloroform but a 2:1 host–guest complexation stoichiometry in the solid state. From single crystal X-ray diffraction, the linear guest molecules thread into cyclic pillar[4]arene[1]quinone host molecules in the solid state, stabilized by CH∙∙∙π interactions and hydrogen bonds. The bromine atoms at the periphery of the guest molecule provide convenience for the further capping of the pseudorotaxanes to construct rotaxanes.
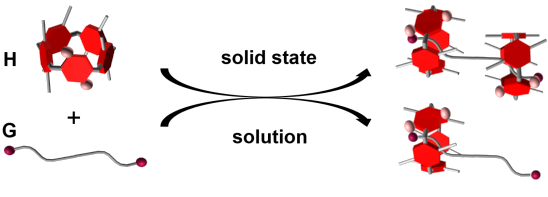
Graphical Abstract
Introduction
Relying on the research of basic science, supramolecular chemistry has become an important mean for constructing functional materials from the bottom up as well as an important way to create new substances with functions [1-5]. Through ingenious designs and the applications of molecular recognition and self-assembly strategies, many exquisite supramolecular architectures have been fabricated, including molecular switches, molecular logic gates, molecular machines, supramolecular polymers, etc. [6-11]. Pseudorotaxanes not only are used as the supramolecular precursors for the synthesis of rotaxanes and catenanes but also play an important role in the construction of supramolecular architectures and chemical topology [12-23]. Seeking new systems to produce pseudorotaxanes is currently considered a “hot topic” in supramolecular chemistry.
As a new class of supramolecular macrocyclic hosts, pillararenes have received extensive attention in recent years due to their unique pillar structures and rich environmental responsiveness [24-29]. There are more and more reports on pillararenes complexing with different guest molecules to construct pseudorotaxanes [30-35]. Previously, our group first demonstrated that alkyl chains can be encapsulated in the pillar[5]arene cavity, forming [2]pseudorotaxanes driven by CH∙∙∙π interactions [36,37]. This discovery facilitated the preparation of threaded structures based on the pillar[5]arene–alkyl chain recognition motif. Benefiting from these results, various compounds with different substituents on the alkyl chains have been used to prepare pseudorotaxanes with pillar[5]arenes and have opened potential applications in different fields [38-43].
So far, fabricating pseudorotaxanes containing more than two components is still a difficult task. Herein, we report new pseudorotaxanes based on the pillar[4]arene[1]quinone H and 1,10-dibromodecane (G, Scheme 1). The pillar[4]arene[1]quinone H, which is composed of four 1,4-diethoxybenzene subunits and one benzoquinone subunit, was prepared by partial oxidation of perethylated pillar[5]arene according to previous reports [44,45]. We found that H and G can be used to build a [3]pseudorotaxane in the solid state but a [2]pseudorotaxane in solution.
![[1860-5397-16-245-i1]](/bjoc/content/inline/1860-5397-16-245-i1.png?scale=2.0&max-width=1024&background=FFFFFF)
Scheme 1: Chemical structures and schematic representation of (a) the pillar[4]arene[1]quinone H; (b) 1,10-dibromodecane (G); and (c) schematic representation of the pseudorotaxanes based on H and G.
Scheme 1: Chemical structures and schematic representation of (a) the pillar[4]arene[1]quinone H; (b) 1,10-di...
Results and Discussion
Host–guest complexation in the solid state
Cocrystals of H and G were obtained by slow evaporation of the solution in methanol. The X-ray crystallography revealed that two host molecules complex one guest molecule, forming a [3]pseudorotaxane in the solid state (Figure 1). In the crystal structure, the alkyl chain of the guest is threaded through the cavities of two host molecules, which is stabilized by multiple CH∙∙∙π interactions and hydrogen bonds. Specifically, there are four hydrogen atoms on the guest molecule to form multiple CH∙∙∙π interactions with the 1,4-diethoxybenzene subunits of the host. In addition, the two bromine atoms at the periphery of the guest molecule are outside the host cavities and form moderate hydrogen bonds with the hydrogen atoms on the ethoxy groups. It is worth mentioning that we did not find any interaction of the benzoquinone subunit of the host with the guest molecule.
![[1860-5397-16-245-1]](/bjoc/content/figures/1860-5397-16-245-1.png?scale=2.0&max-width=1024&background=FFFFFF)
Figure 1: Crystal structures of the [3]pseudorotaxane composed of H and G in the solid state. Color code: C, gray; Br, orange; O, red; and H, white. CH∙∙∙π distances (Å): 2.778, 2.886, 2.778, and 2.886; CH···Br distances (Å): 2.925 and 2.925.
Figure 1: Crystal structures of the [3]pseudorotaxane composed of H and G in the solid state. Color code: C, ...
Host–guest complexation in solution
In order to further study the host–guest binding properties of H and G, we explored the complexation in solution by 1H NMR spectroscopy. As depicted in Figure 2, after the addition of 1.0 equiv of G to a solution of H, all protons on G shifted upfield, which indicated the threading of the alkyl part of the guest into the electron-rich cavity of the host, confirming the complexation between H and G.
![[1860-5397-16-245-2]](/bjoc/content/figures/1860-5397-16-245-2.png?scale=2.0&max-width=1024&background=FFFFFF)
Figure 2: 1H NMR spectra (500 MHz, CDCl3, 298 K): (a) 6.00 mM G; (b) 3.00 mM G + 3.00 mM H; and (c) 6.00 mM H.
Figure 2: 1H NMR spectra (500 MHz, CDCl3, 298 K): (a) 6.00 mM G; (b) 3.00 mM G + 3.00 mM H; and (c) 6.00 mM H....
Matrix-assisted laser desorption/ionization time-of-flight mass spectrometry (MALDI–TOF MS) was conducted to investigate the complexation properties. However, no signal related to the complex but only peaks of H were found, implying weak host–guest interactions between H and G (Figures S3 and S4, Supporting Information File 1).
A Job plot based on the proton NMR data was made to determine the complexation stoichiometry between H and G. The formation signified a 1:1 binding stoichiometry in chloroform-d at room temperature (Figures S5 and S6, Supporting Information File 1). Combined with the mass spectrometry results, we believed that the differences between the stoichiometric ratio of the complexation in the solid state and in solution could be owing to the competitive role of solvent molecules in the combination of H and G. The association constant (Ka) calculated by the nonlinear curve fitting method was 20.0 ± 2.4 M−1 (Figures S7 and S8, Supporting Information File 1), which agreed well with the inference above.
To further explore the geometry of the complexation in solution, we performed a NOESY study (Figure 3). Only one correlative peak was observed between the protons H1 of H and Ha of G, which agreed with the formation of the complexation; the alkyl chains of G were encapsulated in the cavity of H. This further indicated that the interactions between H and G were weak.
![[1860-5397-16-245-3]](/bjoc/content/figures/1860-5397-16-245-3.png?scale=2.0&max-width=1024&background=FFFFFF)
Figure 3: NOESY spectrum of a solution of H and G (500 MHz, chloroform-d, 298 K).
Figure 3: NOESY spectrum of a solution of H and G (500 MHz, chloroform-d, 298 K).
UV–vis absorption properties of the complex in solution
Based on the UV–vis properties of H, we wondered the influence of G on the optical properties of H, so an UV–vis spectroscopy experiment was carried out. We investigated the changes in the UV–vis absorption of the complex at different guest concentrations corresponding to 0.5 and 1 equiv. As shown in Figure 4, the concentration of G did not affect the absorption maximum. This also indicated that the interactions between H and G were weak.
![[1860-5397-16-245-4]](/bjoc/content/figures/1860-5397-16-245-4.png?scale=2.0&max-width=1024&background=FFFFFF)
Figure 4: Normalized UV–vis spectra: H (black); H upon adding 0.5 equiv of G (red); and H upon adding 1 equiv of G (blue). [H] = 3.00 mM.
Figure 4: Normalized UV–vis spectra: H (black); H upon adding 0.5 equiv of G (red); and H upon adding 1 equiv...
Conclusion
In summary, we constructed novel pseudorotaxanes based on a pillar[4]arene[1]quinone and 1,10-dibromodecane. Single crystal X-ray diffraction analysis showed an alkane molecule threaded into the cavities of two pillar[4]arene[1]quinone molecules, forming a [3]pseudorotaxane in the solid state. However, 1H NMR experiments revealed that the pillar[4]arene[1]quinone encapsulated the guest molecule with a 1:1 stoichiometry to form a [2]pseudorotaxane in solution. One possible reason may be that the interactions between H and G were weak and that there was a complexation competition with solvent molecules. Furthermore, the addition of G did not change the maximum UV–vis absorption wavelength of H. The bromine atoms at the periphery of the guest molecule provide convenience for the further capping of the pseudorotaxanes to construct rotaxanes, which will broaden the range of potential applications of pillararene derivatives for the manufacture of sophisticated supramolecular architectures and functional supramolecular systems.
Supporting Information
Supporting Information File 1: Materials and methods, characterizations of H and G, crystallographic data, and characterization studies on the complexation between H and G in solution. | ||
Format: PDF | Size: 811.4 KB | Download |
Supporting Information File 2: CIF file for the complex between H and G. | ||
Format: CIF | Size: 1.3 MB | Download |
References
-
Sun, Q.-F.; Iwasa, J.; Ogawa, D.; Ishido, Y.; Sato, S.; Ozeki, T.; Sei, Y.; Yamaguchi, K.; Fujita, M. Science 2010, 328, 1144–1147. doi:10.1126/science.1188605
Return to citation in text: [1] -
Aida, T.; Meijer, E. W.; Stupp, S. I. Science 2012, 335, 813–817. doi:10.1126/science.1205962
Return to citation in text: [1] -
Chifotides, H. T.; Dunbar, K. R. Acc. Chem. Res. 2013, 46, 894–906. doi:10.1021/ar300251k
Return to citation in text: [1] -
Mattia, E.; Otto, S. Nat. Nanotechnol. 2015, 10, 111–119. doi:10.1038/nnano.2014.337
Return to citation in text: [1] -
Kaphan, D. M.; Levin, M. D.; Bergman, R. G.; Raymond, K. N.; Toste, F. D. Science 2015, 350, 1235–1238. doi:10.1126/science.aad3087
Return to citation in text: [1] -
Zhang, X.; Wang, C. Chem. Soc. Rev. 2011, 40, 94–101. doi:10.1039/b919678c
Return to citation in text: [1] -
Mura, S.; Nicolas, J.; Couvreur, P. Nat. Mater. 2013, 12, 991–1003. doi:10.1038/nmat3776
Return to citation in text: [1] -
Wang, X.; Han, Y.; Liu, Y.; Zou, G.; Gao, Z.; Wang, F. Angew. Chem., Int. Ed. 2017, 56, 12466–12470. doi:10.1002/anie.201704294
Return to citation in text: [1] -
Han, Y.; Tian, Y.; Li, Z.; Wang, F. Chem. Soc. Rev. 2018, 47, 5165–5176. doi:10.1039/c7cs00802c
Return to citation in text: [1] -
Liu, Y.; Zhao, W.; Chen, C.-H.; Flood, A. H. Science 2019, 365, 159–161.
Return to citation in text: [1] -
Zhang, Z.; Cheng, L.; Zhao, J.; Wang, L.; Liu, K.; Yu, W.; Yan, X. Angew. Chem., Int. Ed. 2020, 59, 12139–12146. doi:10.1002/anie.202004152
Return to citation in text: [1] -
Niu, Z.; Gibson, H. W. Chem. Rev. 2009, 109, 6024–6046. doi:10.1021/cr900002h
Return to citation in text: [1] -
Niu, Z.; Huang, F.; Gibson, H. W. J. Am. Chem. Soc. 2011, 133, 2836–2839. doi:10.1021/ja110384v
Return to citation in text: [1] -
Guo, D.-S.; Wang, K.; Wang, Y.-X.; Liu, Y. J. Am. Chem. Soc. 2012, 134, 10244–10250. doi:10.1021/ja303280r
Return to citation in text: [1] -
Langton, M. J.; Beer, P. D. Acc. Chem. Res. 2014, 47, 1935–1949. doi:10.1021/ar500012a
Return to citation in text: [1] -
Qu, D.-H.; Wang, Q.-C.; Zhang, Q.-W.; Ma, X.; Tian, H. Chem. Rev. 2015, 115, 7543–7588. doi:10.1021/cr5006342
Return to citation in text: [1] -
Li, S.-H.; Zhang, H.-Y.; Xu, X.; Liu, Y. Nat. Commun. 2015, 6, No. 7590. doi:10.1038/ncomms8590
Return to citation in text: [1] -
Eichstaedt, K.; Jaramillo-Garcia, J.; Leigh, D. A.; Marcos, V.; Pisano, S.; Singleton, T. A. J. Am. Chem. Soc. 2017, 139, 9376–9381. doi:10.1021/jacs.7b04955
Return to citation in text: [1] -
De Bo, G.; Dolphijn, G.; McTernan, C. T.; Leigh, D. A. J. Am. Chem. Soc. 2017, 139, 8455–8457. doi:10.1021/jacs.7b05640
Return to citation in text: [1] -
Wang, Y.; Sun, J.; Liu, Z.; Nassar, M. S.; Botros, Y. Y.; Stoddart, J. F. Chem. Sci. 2017, 8, 2562–2568. doi:10.1039/c6sc05035b
Return to citation in text: [1] -
Lewis, J. E. M.; Beer, P. D.; Loeb, S. J.; Goldup, S. M. Chem. Soc. Rev. 2017, 46, 2577–2591. doi:10.1039/c7cs00199a
Return to citation in text: [1] -
Roberts, D. A.; Pilgrim, B. S.; Nitschke, J. R. Chem. Soc. Rev. 2018, 47, 626–644. doi:10.1039/c6cs00907g
Return to citation in text: [1] -
Li, G.; Wang, L.; Wu, L.; Guo, Z.; Zhao, J.; Liu, Y.; Bai, R.; Yan, X. J. Am. Chem. Soc. 2020, 142, 14343–14349. doi:10.1021/jacs.0c06416
Return to citation in text: [1] -
Ogoshi, T.; Kanai, S.; Fujinami, S.; Yamagishi, T.-a.; Nakamoto, Y. J. Am. Chem. Soc. 2008, 130, 5022–5023. doi:10.1021/ja711260m
Return to citation in text: [1] -
Cao, D.; Kou, Y.; Liang, J.; Chen, Z.; Wang, L.; Meier, H. Angew. Chem., Int. Ed. 2009, 48, 9721–9723. doi:10.1002/anie.200904765
Return to citation in text: [1] -
Xue, M.; Yang, Y.; Chi, X.; Yan, X.; Huang, F. Chem. Rev. 2015, 115, 7398–7501. doi:10.1021/cr5005869
Return to citation in text: [1] -
Ogoshi, T.; Yamagishi, T.-a.; Nakamoto, Y. Chem. Rev. 2016, 116, 7937–8002. doi:10.1021/acs.chemrev.5b00765
Return to citation in text: [1] -
Han, C.; Zhao, D.; Li, H.; Wang, H.; Huang, X.; Sun, D. ChemistrySelect 2018, 3, 11–14. doi:10.1002/slct.201702793
Return to citation in text: [1] -
Jie, K.; Zhou, Y.; Li, E.; Huang, F. Acc. Chem. Res. 2018, 51, 2064–2072. doi:10.1021/acs.accounts.8b00255
Return to citation in text: [1] -
Si, W.; Chen, L.; Hu, X.-B.; Tang, G.; Chen, Z.; Hou, J.-L.; Li, Z.-T. Angew. Chem., Int. Ed. 2011, 50, 12564–12568. doi:10.1002/anie.201106857
Return to citation in text: [1] -
Chen, Y.; Cao, D.; Wang, L.; He, M.; Zhou, L.; Schollmeyer, D.; Meier, H. Chem. – Eur. J. 2013, 19, 7064–7070. doi:10.1002/chem.201204628
Return to citation in text: [1] -
Xia, B.; Xue, M. Chem. Commun. 2014, 50, 1021–1023. doi:10.1039/c3cc48014c
Return to citation in text: [1] -
Chen, H.; Fan, J.; Hu, X.; Ma, J.; Wang, S.; Li, J.; Yu, Y.; Jia, X.; Li, C. Chem. Sci. 2015, 6, 197–202. doi:10.1039/c4sc02422b
Return to citation in text: [1] -
Sun, Y.; Fu, W.; Chen, C.; Wang, J.; Yao, Y. Chem. Commun. 2017, 53, 3725–3728. doi:10.1039/c7cc00291b
Return to citation in text: [1] -
Du, X.-S.; Wang, C.-Y.; Jia, Q.; Deng, R.; Tian, H.-S.; Zhang, H.-Y.; Meguellati, K.; Yang, Y.-W. Chem. Commun. 2017, 53, 5326–5329. doi:10.1039/c7cc02364b
Return to citation in text: [1] -
Zhang, Z.; Xia, B.; Han, C.; Yu, Y.; Huang, F. Org. Lett. 2010, 12, 3285–3287. doi:10.1021/ol100883k
Return to citation in text: [1] -
Zhang, Z.; Luo, Y.; Chen, J.; Dong, S.; Yu, Y.; Ma, Z.; Huang, F. Angew. Chem., Int. Ed. 2011, 50, 1397–1401. doi:10.1002/anie.201006693
Return to citation in text: [1] -
Ogoshi, T.; Demachi, K.; Kitajima, K.; Yamagishi, T.-a. Chem. Commun. 2011, 47, 7164–7166. doi:10.1039/c1cc12333e
Return to citation in text: [1] -
Hu, X.-B.; Chen, Z.; Tang, G.; Hou, J.-L.; Li, Z.-T. J. Am. Chem. Soc. 2012, 134, 8384–8387. doi:10.1021/ja302292c
Return to citation in text: [1] -
Ni, M.; Hu, X.-Y.; Jiang, J.; Wang, L. Chem. Commun. 2014, 50, 1317–1319. doi:10.1039/c3cc47823h
Return to citation in text: [1] -
Sun, C.-L.; Xu, J.-F.; Chen, Y.-Z.; Niu, L.-Y.; Wu, L.-Z.; Tung, C.-H.; Yang, Q.-Z. Chin. Chem. Lett. 2015, 26, 843–846. doi:10.1016/j.cclet.2015.05.030
Return to citation in text: [1] -
Ma, J.; Meng, Q.; Hu, X.; Li, B.; Ma, S.; Hu, B.; Li, J.; Jia, X.; Li, C. Org. Lett. 2016, 18, 5740–5743. doi:10.1021/acs.orglett.6b03005
Return to citation in text: [1] -
Li, B.; Meng, Z.; Li, Q.; Huang, X.; Kang, Z.; Dong, H.; Chen, J.; Sun, J.; Dong, Y.; Li, J.; Jia, X.; Sessler, J. L.; Meng, Q.; Li, C. Chem. Sci. 2017, 8, 4458–4464. doi:10.1039/c7sc01438d
Return to citation in text: [1] -
Han, C.; Zhang, Z.; Yu, G.; Huang, F. Chem. Commun. 2012, 48, 9876–9878. doi:10.1039/c2cc35498e
Return to citation in text: [1] -
Ogoshi, T.; Yamafuji, D.; Kotera, D.; Aoki, T.; Fujinami, S.; Yamagishi, T.-a. J. Org. Chem. 2012, 77, 11146–11152. doi:10.1021/jo302283n
Return to citation in text: [1]
1. | Sun, Q.-F.; Iwasa, J.; Ogawa, D.; Ishido, Y.; Sato, S.; Ozeki, T.; Sei, Y.; Yamaguchi, K.; Fujita, M. Science 2010, 328, 1144–1147. doi:10.1126/science.1188605 |
2. | Aida, T.; Meijer, E. W.; Stupp, S. I. Science 2012, 335, 813–817. doi:10.1126/science.1205962 |
3. | Chifotides, H. T.; Dunbar, K. R. Acc. Chem. Res. 2013, 46, 894–906. doi:10.1021/ar300251k |
4. | Mattia, E.; Otto, S. Nat. Nanotechnol. 2015, 10, 111–119. doi:10.1038/nnano.2014.337 |
5. | Kaphan, D. M.; Levin, M. D.; Bergman, R. G.; Raymond, K. N.; Toste, F. D. Science 2015, 350, 1235–1238. doi:10.1126/science.aad3087 |
30. | Si, W.; Chen, L.; Hu, X.-B.; Tang, G.; Chen, Z.; Hou, J.-L.; Li, Z.-T. Angew. Chem., Int. Ed. 2011, 50, 12564–12568. doi:10.1002/anie.201106857 |
31. | Chen, Y.; Cao, D.; Wang, L.; He, M.; Zhou, L.; Schollmeyer, D.; Meier, H. Chem. – Eur. J. 2013, 19, 7064–7070. doi:10.1002/chem.201204628 |
32. | Xia, B.; Xue, M. Chem. Commun. 2014, 50, 1021–1023. doi:10.1039/c3cc48014c |
33. | Chen, H.; Fan, J.; Hu, X.; Ma, J.; Wang, S.; Li, J.; Yu, Y.; Jia, X.; Li, C. Chem. Sci. 2015, 6, 197–202. doi:10.1039/c4sc02422b |
34. | Sun, Y.; Fu, W.; Chen, C.; Wang, J.; Yao, Y. Chem. Commun. 2017, 53, 3725–3728. doi:10.1039/c7cc00291b |
35. | Du, X.-S.; Wang, C.-Y.; Jia, Q.; Deng, R.; Tian, H.-S.; Zhang, H.-Y.; Meguellati, K.; Yang, Y.-W. Chem. Commun. 2017, 53, 5326–5329. doi:10.1039/c7cc02364b |
24. | Ogoshi, T.; Kanai, S.; Fujinami, S.; Yamagishi, T.-a.; Nakamoto, Y. J. Am. Chem. Soc. 2008, 130, 5022–5023. doi:10.1021/ja711260m |
25. | Cao, D.; Kou, Y.; Liang, J.; Chen, Z.; Wang, L.; Meier, H. Angew. Chem., Int. Ed. 2009, 48, 9721–9723. doi:10.1002/anie.200904765 |
26. | Xue, M.; Yang, Y.; Chi, X.; Yan, X.; Huang, F. Chem. Rev. 2015, 115, 7398–7501. doi:10.1021/cr5005869 |
27. | Ogoshi, T.; Yamagishi, T.-a.; Nakamoto, Y. Chem. Rev. 2016, 116, 7937–8002. doi:10.1021/acs.chemrev.5b00765 |
28. | Han, C.; Zhao, D.; Li, H.; Wang, H.; Huang, X.; Sun, D. ChemistrySelect 2018, 3, 11–14. doi:10.1002/slct.201702793 |
29. | Jie, K.; Zhou, Y.; Li, E.; Huang, F. Acc. Chem. Res. 2018, 51, 2064–2072. doi:10.1021/acs.accounts.8b00255 |
12. | Niu, Z.; Gibson, H. W. Chem. Rev. 2009, 109, 6024–6046. doi:10.1021/cr900002h |
13. | Niu, Z.; Huang, F.; Gibson, H. W. J. Am. Chem. Soc. 2011, 133, 2836–2839. doi:10.1021/ja110384v |
14. | Guo, D.-S.; Wang, K.; Wang, Y.-X.; Liu, Y. J. Am. Chem. Soc. 2012, 134, 10244–10250. doi:10.1021/ja303280r |
15. | Langton, M. J.; Beer, P. D. Acc. Chem. Res. 2014, 47, 1935–1949. doi:10.1021/ar500012a |
16. | Qu, D.-H.; Wang, Q.-C.; Zhang, Q.-W.; Ma, X.; Tian, H. Chem. Rev. 2015, 115, 7543–7588. doi:10.1021/cr5006342 |
17. | Li, S.-H.; Zhang, H.-Y.; Xu, X.; Liu, Y. Nat. Commun. 2015, 6, No. 7590. doi:10.1038/ncomms8590 |
18. | Eichstaedt, K.; Jaramillo-Garcia, J.; Leigh, D. A.; Marcos, V.; Pisano, S.; Singleton, T. A. J. Am. Chem. Soc. 2017, 139, 9376–9381. doi:10.1021/jacs.7b04955 |
19. | De Bo, G.; Dolphijn, G.; McTernan, C. T.; Leigh, D. A. J. Am. Chem. Soc. 2017, 139, 8455–8457. doi:10.1021/jacs.7b05640 |
20. | Wang, Y.; Sun, J.; Liu, Z.; Nassar, M. S.; Botros, Y. Y.; Stoddart, J. F. Chem. Sci. 2017, 8, 2562–2568. doi:10.1039/c6sc05035b |
21. | Lewis, J. E. M.; Beer, P. D.; Loeb, S. J.; Goldup, S. M. Chem. Soc. Rev. 2017, 46, 2577–2591. doi:10.1039/c7cs00199a |
22. | Roberts, D. A.; Pilgrim, B. S.; Nitschke, J. R. Chem. Soc. Rev. 2018, 47, 626–644. doi:10.1039/c6cs00907g |
23. | Li, G.; Wang, L.; Wu, L.; Guo, Z.; Zhao, J.; Liu, Y.; Bai, R.; Yan, X. J. Am. Chem. Soc. 2020, 142, 14343–14349. doi:10.1021/jacs.0c06416 |
6. | Zhang, X.; Wang, C. Chem. Soc. Rev. 2011, 40, 94–101. doi:10.1039/b919678c |
7. | Mura, S.; Nicolas, J.; Couvreur, P. Nat. Mater. 2013, 12, 991–1003. doi:10.1038/nmat3776 |
8. | Wang, X.; Han, Y.; Liu, Y.; Zou, G.; Gao, Z.; Wang, F. Angew. Chem., Int. Ed. 2017, 56, 12466–12470. doi:10.1002/anie.201704294 |
9. | Han, Y.; Tian, Y.; Li, Z.; Wang, F. Chem. Soc. Rev. 2018, 47, 5165–5176. doi:10.1039/c7cs00802c |
10. | Liu, Y.; Zhao, W.; Chen, C.-H.; Flood, A. H. Science 2019, 365, 159–161. |
11. | Zhang, Z.; Cheng, L.; Zhao, J.; Wang, L.; Liu, K.; Yu, W.; Yan, X. Angew. Chem., Int. Ed. 2020, 59, 12139–12146. doi:10.1002/anie.202004152 |
44. | Han, C.; Zhang, Z.; Yu, G.; Huang, F. Chem. Commun. 2012, 48, 9876–9878. doi:10.1039/c2cc35498e |
45. | Ogoshi, T.; Yamafuji, D.; Kotera, D.; Aoki, T.; Fujinami, S.; Yamagishi, T.-a. J. Org. Chem. 2012, 77, 11146–11152. doi:10.1021/jo302283n |
38. | Ogoshi, T.; Demachi, K.; Kitajima, K.; Yamagishi, T.-a. Chem. Commun. 2011, 47, 7164–7166. doi:10.1039/c1cc12333e |
39. | Hu, X.-B.; Chen, Z.; Tang, G.; Hou, J.-L.; Li, Z.-T. J. Am. Chem. Soc. 2012, 134, 8384–8387. doi:10.1021/ja302292c |
40. | Ni, M.; Hu, X.-Y.; Jiang, J.; Wang, L. Chem. Commun. 2014, 50, 1317–1319. doi:10.1039/c3cc47823h |
41. | Sun, C.-L.; Xu, J.-F.; Chen, Y.-Z.; Niu, L.-Y.; Wu, L.-Z.; Tung, C.-H.; Yang, Q.-Z. Chin. Chem. Lett. 2015, 26, 843–846. doi:10.1016/j.cclet.2015.05.030 |
42. | Ma, J.; Meng, Q.; Hu, X.; Li, B.; Ma, S.; Hu, B.; Li, J.; Jia, X.; Li, C. Org. Lett. 2016, 18, 5740–5743. doi:10.1021/acs.orglett.6b03005 |
43. | Li, B.; Meng, Z.; Li, Q.; Huang, X.; Kang, Z.; Dong, H.; Chen, J.; Sun, J.; Dong, Y.; Li, J.; Jia, X.; Sessler, J. L.; Meng, Q.; Li, C. Chem. Sci. 2017, 8, 4458–4464. doi:10.1039/c7sc01438d |
36. | Zhang, Z.; Xia, B.; Han, C.; Yu, Y.; Huang, F. Org. Lett. 2010, 12, 3285–3287. doi:10.1021/ol100883k |
37. | Zhang, Z.; Luo, Y.; Chen, J.; Dong, S.; Yu, Y.; Ma, Z.; Huang, F. Angew. Chem., Int. Ed. 2011, 50, 1397–1401. doi:10.1002/anie.201006693 |
© 2020 Sheng et al.; licensee Beilstein-Institut.
This is an Open Access article under the terms of the Creative Commons Attribution License (https://creativecommons.org/licenses/by/4.0). Please note that the reuse, redistribution and reproduction in particular requires that the author(s) and source are credited and that individual graphics may be subject to special legal provisions.
The license is subject to the Beilstein Journal of Organic Chemistry terms and conditions: (https://www.beilstein-journals.org/bjoc/terms)