Abstract
The synthesis of complex cyclic compounds is extremely challenging for organic chemists. Many transition-metal-salt-mediated cyclizations are reported in literature. Hg(II) salts have been successfully employed in cyclizations to form complex heterocyclic and carbocyclic structures that are impossible to synthesize with other transition metal salts. In this review, we have summarized cyclization reactions that are performed with Hg(II) salts. These salts are also successfully applied in stoichiometric or catalytic amounts to form complex cyclic structures and natural products.
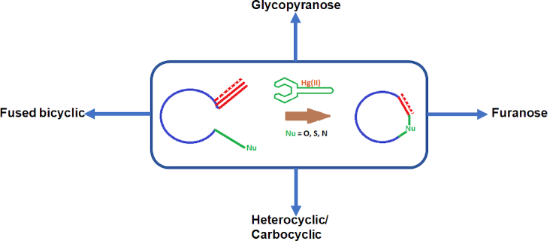
Graphical Abstract
Introduction
The use of transition metal reagents has found considerable applications in organic synthesis [1-4] and has radically changed the realm of chemical science. It also provides a powerful tool for the construction of complex molecular frameworks [5-7]. A plethora of reviews involving transition metals such as Pd(II) [8-10], Ru(II) [11-13], Rh(III) [14-16], Mn(II) [17-19], Au(II/I) [20-22], Ag(I) [23-25] etc. in both cascade and sequential reactions have been published. Electrophilic Hg(II) salts are important reagents in organic synthesis and there is published literature establishing this fact [26-29]. However, the main drawback of Hg(II) salts, as compared to other transition metal salts, is their increased toxicity [30,31]. Hg(II) salts on the other hand, are very cheap in comparison to other transition metal salts (Table 1) and one of the soft Lewis acids of the periodic table [32]. Hg(II) salts have already manifested some unique reactivity and therefore attracted increasing interest from chemists [33]. Many examples involving Hg(II) salts with unsaturated bonds in presence of various nucleophiles giving rise to various types of products are abound in the literature. Utilization of Hg(II) salts in the intramolecular cationic cyclization of olefinic, acetylenic, and allenic substrates having aromatic rings, nucleophiles, and heteroatoms in the neighborhood were well documented. Hg(II) reagents were also often employed in the important cyclization step during total syntheses of many natural products [34]. Despite its wide application in ring formation reactions, only a few review articles on Hg(II)-salt-mediated cyclization reactions are available in the literature [35]. This review describes the intramolecular cyclization of unsaturated compounds in the presence of stoichiometric/catalytic amounts of Hg(II) salts. The classification of this review is based on the following topics.
- Cyclization reactions involving the stoichiometric amount of Hg(II) salts.
- Cyclization reactions involving the catalytic amount of Hg(II) salts.
- Total synthesis involving Hg(II)-salt-mediated cyclization reactions.
Table 1: Comparison of price of reagent grade Hg(II) salts with other transition metal salts.
Name of salts | CAS No. | Price $/ga |
mercury(II) chloride HgCl2 | 7487-94-7 | 0.67b |
mercury(II) acetate Hg(OAc)2 | 1600-27-7 | 1.35b |
mercuric triflate Hg(OTf)2 | 49540-00-3 | 12.7c |
mercury(II) perchlorate Hg(ClO4)2 | 304656-34-6 | 2.83c |
auric chloride AuCl3 | 13453-07-1 | 112d |
silver perchlorate AgClO4 | 7783-93-9 | 8.24c |
platinum chloride PtCl2 | 10025-65-7 | 115d |
aPrices are from http://www.sigmaaldrich.com (as on 05-08-2021). b100 g, c25 g, d5 g pack size.
The generalized mechanism for cyclization reactions are, alkenes/alkynes 1 initially react with Hg(II) salts (HgX2) leading to the formation of a mercurial carbonium ion 2 followed by the attack of an intramolecular nucleophile giving rise to a cyclized mercuro–halide complex compound 3 (Scheme 1).
Scheme 1: Schematic representation of Hg(II)-mediated addition to an unsaturated bond.
Scheme 1: Schematic representation of Hg(II)-mediated addition to an unsaturated bond.
Review
Cyclization reactions involving stoichiometric amounts of Hg(II) salts
Cyclization involving alkenes (>C=C<)
In 1900 and 1901, the Sand and Biilmann group had first reported the Hg(II)-mediated cyclization of allyl alcohol using Hg(II) nitrate (Hg(NO3)2) in two separate publications [36,37]. The cyclized product thus formed was further treated with iodine to get trans-2,5-bis(iodomethyl)-p-dioxane (4a). The formation of trans-isomer 4a as the major product and cis-isomer 4b as a minor product was later confirmed by Summerbell et al. by repeating the same experiments (Scheme 2) [38].
Scheme 2: First report of Hg(II)-mediated synthesis of 2,5-dioxane derivatives from allyl alcohol.
Scheme 2: First report of Hg(II)-mediated synthesis of 2,5-dioxane derivatives from allyl alcohol.
Cyclization of biallyl ether 5 in presence of mercuric acetate (Hg(OAc)2) in an aqueous medium was reported to synthesize a diastereomeric mixture of 2,6-disubstituted-p-dioxane 6. The outcome of the reaction was much generalized with no detailed discussion about the ratio of diastereomeric products [39]. Later, Summerbell and co-workers modified the reaction conditions to synthesize 2,6-disubstituted dioxane derivatives 6 (cis/trans 16:1). Unlike 2,5-disubstituted dioxane derivatives, here the cis-isomer was the major product. A higher ratio of the cis-dioxane 6 was achieved by increasing the reaction time and acidity of the reaction medium, while elevated temperature showed no effect (Scheme 3) [40].
Scheme 3: Stepwise synthesis of 2,6-distubstituted dioxane derivatives.
Scheme 3: Stepwise synthesis of 2,6-distubstituted dioxane derivatives.
The mercuricyclization was also employed in the field of carbohydrate chemistry for the synthesis of α-ᴅ-C-glycopyranosyl derivatives. The reaction between carbohydrate alkene precursor 7 and Hg(OAc)2 proceeds with high stereoselectivity to give the α-ᴅ-C-glycopyranosyl derivative (1,5-trans-isomer) 8 as a single isomer [41]. Treatment of compound 8 with sodium borohydride (NaBH4) under phase transfer conditions (PTC) yields compound 9 as the only product. The selectivity of α-stereochemistry was primarily due to the strong directing effect of the neighboring benzyl ether group with the Hg(OAc)2. When cyclic mercuric halide 8 was treated with NaBH4 and oxygen (O2) in DMF oxidative demercuration takes place to give alcohol 10 in quantitative yield (Scheme 4).
Scheme 4: Cyclization of carbohydrate alkene precursor.
Scheme 4: Cyclization of carbohydrate alkene precursor.
The methodology utilized in Scheme 5 had been successfully employed for the preparation of C-glycopyranosyl derivatives of common and uncommon sugars like α-gluco, α-manno, β-altro, β-ido, α- and β-gulo, β-talo, α-galacto, and α-allo C-glycopyranosides [42]. Vinylated derivatives of aldopentoses 11 were treated with Hg(OAc)2 affording the corresponding cyclized pyanosylmethylmercuric chloride derivatives 12 (Scheme 5). This methodology can be used to synthesize rare C-glycosyl carbohydrates from easily available sugars.
Scheme 5: Hg(II)-mediated synthesis of C-glucopyranosyl derivatives.
Scheme 5: Hg(II)-mediated synthesis of C-glucopyranosyl derivatives.
In a similar manner, stereoselective cyclization of C-glycosyl amino acid derivative 13 using mercuric trifluoroacetate Hg(TFA)2 at room temperature was performed. The reaction proceeds primarily through stereoselective cyclization to give α-ᴅ-C-glycopyranosyl amino acid derivative 14 as the major product [43]. Nevertheless, C-mannopyranosyl derivatives cannot be achieved in a similar manner as reductive elimination forms during the mercury removal process (Scheme 6).
Scheme 6: Synthesis of C-glycosyl amino acid derivative using Hg(TFA)2.
Scheme 6: Synthesis of C-glycosyl amino acid derivative using Hg(TFA)2.
Mercury(II) salts had been effectively used to synthesize five-membered furanose derivatives with high stereoselectivity. Nicotra et al. developed Hg(OAc)2-mediated cyclization of hydroxy-alkene derivative 15 to form α-ᴅ-ribose derivative 16 at room temperature (Scheme 7). They had confirmed the formation of the α-isomer of ᴅ-C-ribofuranosyl 16 predominantly over the β-isomer (α/β 95:5) [44].
Scheme 7: Hg(OAc)2-mediated synthesis of α-ᴅ-ribose derivative.
Scheme 7: Hg(OAc)2-mediated synthesis of α-ᴅ-ribose derivative.
In contrast, when Reitz and co-workers carried out a ring formation of benzylated C-arabinofuranoside derivative 17 in presence of 1.4 equiv of Hg(OAc)2 and sodium bromide (NaBr) at room temperature, then β-ᴅ-arabinose derivative 18 was formed as the major product (Scheme 8) [45]. The high stereoselectivity of β-derivative 18 at the anomeric position was predominantly due to the presence of the benzyl groups at the C-2 and C-3 positions of the starting material.
Scheme 8: Synthesis of β-ᴅ-arabinose derivative 18.
Scheme 8: Synthesis of β-ᴅ-arabinose derivative 18.
It had been cited in many publications that the stereoselectivity of products formed due to Hg(II)-salt-mediated cyclization reactions of alkene-alcohol derivatives depends on several factors: the nature of the Hg(II) salts [46], the starting materials [47], and the effect of H2O (protic solvent) in the reaction media [48].
Pulido et al. had developed a conversion of allylsilyl alcohols 19 to diastereomeric mixtures of tetrahydrofuran derivatives 20A and 20B (Scheme 9) [47]. It was reported that more substituted alkyl groups present in allylsilyl alcohol 20b direct the selective formation of diastereomeric products. They also observed that changes in Hg(II) salts result in different ratios of the cis- and trans-isomer in the cyclized products [47]. The differences in cis and trans ratios were primarily due to the basicity of anions associated with Hg(II) salts [46].
Scheme 9: Hg(OAc)2-mediated synthesis of tetrahydrofuran derivatives.
Scheme 9: Hg(OAc)2-mediated synthesis of tetrahydrofuran derivatives.
A similar type of reaction methodology was employed for the formation of a bicyclic nucleoside analog. 4'-C-vinylribofuranoside derivative 21 on treatment with Hg(TFA)2 followed by reduction with NaBH4 leads to the formation of bicyclic nucleoside derivative 22 (Scheme 10) [49].
Scheme 10: Synthesis of Hg(TFA)2-mediated bicyclic nucleoside derivative.
Scheme 10: Synthesis of Hg(TFA)2-mediated bicyclic nucleoside derivative.
Pyrrolidine and piperidine derivatives were also synthesized by Hg(II)-salt-induced cyclization. N-Isopropyl-1-aminohex-4-ene (23) on treatment with 1 equiv HgCl2 followed by reduction with NaBH4 yielded pyrrolidine 24 and piperidine derivative 25 in the ratio of 7:3 [50]. N-Methylaniline derivative 26 undergoes cyclization with HgCl2 and gave cis-mercuro chlorides 27 and 28 as isolated products (Scheme 11) [51].
Scheme 11: Synthesis of pyrrolidine and piperidine derivatives.
Scheme 11: Synthesis of pyrrolidine and piperidine derivatives.
For the synthesis of diastereomeric pyrrolidine derivatives, a Hg(II) salt had been used. When HgCl2 was added to secondary methylamine derivatives 29 and 31 followed by reduction with NaBH4 a mixture of diastereomers 30a,b and 32a,b was obtained, respectively. trans-Isomers were formed as major products over cis-isomers (Scheme 12) [52].
Scheme 12: HgCl2-mediated synthesis of diastereomeric pyrrolidine derivatives.
Scheme 12: HgCl2-mediated synthesis of diastereomeric pyrrolidine derivatives.
Five- and six-membered N-containing heterocyclic phosphonates were synthesized by intramolecular cyclization of alkenyl α-aminophosphonates in a similar way with the treatment of Hg(OAc)2 [53]. The cyclized products 34A,B formed from starting material 33 were regiospecific and followed Markovnikoff’s type addition in the reaction [54-57]. It was also reported that the formation of α-phosphorylated pyrrolidines mostly takes place in regio- and stereoselective ways depending on the reaction conditions (Scheme 13) [53,58].
Scheme 13: HgCl2-mediated cyclization of alkenyl α-aminophosphonates.
Scheme 13: HgCl2-mediated cyclization of alkenyl α-aminophosphonates.
Cyclization of 4-cycloocten-1-ol (35) with Hg(OAc)2 resulted in formation of two types of fused bicyclic products, 9-oxabicyclo[3.3.1]nonane (36) and 9-oxabicyclo[4.2.1]nonane (37) (Scheme 14) [59,60]. It was observed that the regioselective synthesis of 9-oxabicyclo[4.2.1]nonane (37) was favored when NaBH4 and sodium acetate NaOAc were present, while in absence of NaOAc, 9-oxabicyclo[3.3.1]nonane (36) was formed as an exclusive product.
Scheme 14: Cyclization of 4-cycloocten-1-ol with Hg(OAc)2 forming fused bicyclic products.
Scheme 14: Cyclization of 4-cycloocten-1-ol with Hg(OAc)2 forming fused bicyclic products.
In a similar manner, an aminomercuration reaction had been successfully employed in the cyclization of trans-amino alcohol 38 leading to the formation of (1R,2R,6R)-9-benzyl-9-azabicyclo[4.2.1]nonan-2-ol (39). The bicyclic derivative 39 through the number of consequent reactions formed a highly potent (+)/(−)-pyrido[3,4-b]homotropane (40), a bridged nicotinoid (Scheme 15) [61].
Scheme 15: trans-Amino alcohol formation through Hg(II)-salt-mediated cyclization.
Scheme 15: trans-Amino alcohol formation through Hg(II)-salt-mediated cyclization.
Similarly, precursor 41 at room temperature in the presence of Hg(OAc)2 (1 equiv) in CH2Cl2 cyclized to form 2-aza- or 2-oxabicyclic mercuri derivatives. Further, treatment of intermediates with NaBH4 (4 equiv) in presence of 5% aq NaOH solution for 1 hour at room temperature produced bicyclic derivatives 42 (Scheme 16) [62]. The diasteroselectivity of the products formed depends on the heteroatoms involved; azamercuration yields more selectivity than oxymercuration.
Scheme 16: Hg(OAc)2-mediated 2-aza- or 2-oxa-bicyclic ring formations.
Scheme 16: Hg(OAc)2-mediated 2-aza- or 2-oxa-bicyclic ring formations.
Nixon et al. reported a Hg(II)-salt-induced alkenyl hydroperoxide 43 cyclization to synthesize cyclic peroxides 44 as the major product, which on further treatment with NaBH4 gives compound 45 along with the unexpected product 46 (Scheme 17) [63].
Scheme 17: Hg(II)-salt-induced cyclic peroxide formation.
Scheme 17: Hg(II)-salt-induced cyclic peroxide formation.
Later they had reported the reaction between alkenyl hydroperoxide 47 and aldehydes 48 to form hemiperoxyacetal 49 which subsequently reacted with Hg(OAc)2 and a catalytic amount of perchloric acid (HClO4) forming cyclized product 1,2,4-trioxanemercuri bromide 50. The product thus formed was reduced with NaBH4 to form compound 51 (Scheme 18) [64].
Scheme 18: Hg(OAc)2-mediated formation of 1,2,4-trioxanes.
Scheme 18: Hg(OAc)2-mediated formation of 1,2,4-trioxanes.
Kurbanov et al. first reported the cyclization of different isoprenoid derivatives to hexahydrochromene derivatives using Hg(II) salts [65]. They had shown that trans-geranylacetone (52) produces trans-2,5,5,9-tetramethylhexahydrochromene (53) while the cis-cyclized product was formed from cis-isoprenoid derivatives. Later, Hoye et al. used similar reaction conditions to cyclize dienes 54 with 1.1 equiv of Hg(TFA)2 to yield endocyclic enol ether 55 in almost quantitative yield [66]. They had also performed experiments with different isoprenoid derivatives (carboxylic acid, ketones alcohols, and keto esters) to form different bicyclic products (Scheme 19).
Scheme 19: Endocyclic enol ether derivative formation through Hg(II) salts.
Scheme 19: Endocyclic enol ether derivative formation through Hg(II) salts.
A Hg(II)-salt-promoted cyclization of amidal 56 was performed to synthesize optically active cyclic alanine derivatives 57 as 1:1 diastereomeric mixture [67]. Diastereomers were further separated by column chromatography and afforded enantiomerically pure isomers of N-substituted imidazolidine-4-ones 57 (Scheme 20).
Scheme 20: Synthesis of optically active cyclic alanine derivatives.
Scheme 20: Synthesis of optically active cyclic alanine derivatives.
In a similar manner, N-Cbz-protected amine 58 undergoes cyclization using Hg(TFA)2 (1 equiv) and yielded a dinitrogen-containing mixture of diastereomeric alicyclic derivatives 59. The tetrahydropyrimidin-4(1H)-one-mercuri trifluoroacetate derivative 59 on successive treatment with NaBr and LiBH4 gives a mixture of tetrahydropyrimidin-4(1H)-one derivatives 60 in the diastereomeric ratio of 63:37 after separation by column chromatography [68] (Scheme 21).
Scheme 21: Hg(II)-salt-mediated formation of tetrahydropyrimidin-4(1H)-one derivatives.
Scheme 21: Hg(II)-salt-mediated formation of tetrahydropyrimidin-4(1H)-one derivatives.
Mercury(II) salts had also been successfully utilized in the cyclization of ether derivatives 61 to form stereoselectively trans-4,5-disubstituted oxazolidine derivatives 62 (Scheme 22) [69]. Later it was reported, when homologous allyloxycarbamate derivative 63 was cyclized with Hg(TFA)2 then five- and six-membered rings 64 and 65 were formed with a 1:4 ratio (Scheme 22) [70]. The greater yield of the six-membered product was primarily due to the electron-withdrawing effect of ethereal oxygen which in turn destabilizes the carbocation at the β-carbon and hence the nucleophilic attack at the γ-carbon took place.
Scheme 22: Cyclization of ether derivatives to form stereoselective oxazolidine derivatives.
Scheme 22: Cyclization of ether derivatives to form stereoselective oxazolidine derivatives.
Cyclization of amide derivative 66 induced by Hg(OAc)2 followed by reduction with LiBH4 afforded a mixture of compounds 67A/67B [71]. The formation of endo,trans-product as a major product over the exo,cis-isomer was primarily due to a stereoinduction effect (Scheme 23). The small amount of exo,cis-isomers 67Ba/67Bc was only detected by HPLC analysis of the crude reaction mixture.
Scheme 23: Cyclization of amide derivatives induced by Hg(OAc)2.
Scheme 23: Cyclization of amide derivatives induced by Hg(OAc)2.
Takacs et al. observed the rapid cyclization of salicylamide-derived amidal auxiliary derivatives 68 and 70 in presence of 1.5 equiv of a 1:1 mixture of Hg(OAc)2/Hg(TFA)2 resulting in a diastereomeric pair of cyclized products 69 and 71, respectively. It was observed that the cis-isomer was predominant in the case of the five-membered ring while the trans-isomer was predominant in the case of the six-membered ring formation (Scheme 24) [72].
Scheme 24: Hg(OAc)2/Hg(TFA)2-promoted cyclization of salicylamide-derived amidal auxiliary derivatives.
Scheme 24: Hg(OAc)2/Hg(TFA)2-promoted cyclization of salicylamide-derived amidal auxiliary derivatives.
Cyclization involving alkynes (-C≡C-)
Hg(II)-salt-mediated cyclizations were also observed in the case of alkynes. Arylalkynes 72 were cyclized via Hg(II)-salt-induced reactions to form benzopyran derivatives 73 [73-75]. Interestingly in the case of 1-aryloxy-4-arylthio-2-butyne derivatives 74, selective ring closure on the oxygen side afforded 6-membered chromene derivatives 75 but no thiochromene derivatives were observed [76]. Compound 75 on refluxing with trifluoroacetic acid isomerized to form exocyclic trans-compound 76. It was reported that 1,4-di(arylthiol)-2-butyne derivatives only afforded usual Hg(II)-salt-mediated hydrated products instead of cyclized thiochromene derivatives. Balasubramanian et al. had performed a mercuric-oxide-mediated cyclization of 1,6-di(aryloxy)-2,4-hexadiyne derivatives 77 to get bichromene derivatives 78 (Scheme 25) [77].
Scheme 25: Hg(II)-salt-mediated cyclization to form dihydrobenzopyrans.
Scheme 25: Hg(II)-salt-mediated cyclization to form dihydrobenzopyrans.
A HgCl2-induced cyclization also takes place for acetylenic silyl enol ether derivative 79 forming carbocyclic compounds 81 with good yields via intermediate 80 [78]. The cyclization of compounds 79 undergo regioselective addition with the triple bond in exocyclic alkene position leading to the formation of α-mercury ketone 80, which were later functionalized by electrophilic addition (Scheme 26).
Scheme 26: HgCl2-induced cyclization of acetylenic silyl enol ether derivatives.
Scheme 26: HgCl2-induced cyclization of acetylenic silyl enol ether derivatives.
Schwartz et al. developed a new route for the synthesis of exocyclic enol ether 83 and endocyclic enol ether 85 involving a Hg(II)-induced cyclization of acetylenic alcohols 82 and 84, respectively [79]. This work revealed that the regioselectivity of the Hg(II) addition to the alkyne may be influenced by diastereomers; in the case of cis-isomer 82 exocyclic enol ether 83 was formed while for trans-isomer 84 the reaction takes place with a much slower rate yielding endocyclic enol ether 85 as the only product (Scheme 27) [79].
Scheme 27: Synthesis of exocyclic and endocyclic enol ether derivatives.
Scheme 27: Synthesis of exocyclic and endocyclic enol ether derivatives.
trans-Acetylenic alcohol 86 on treatment with HgCl2 (0.5 equiv) in presence of N-bromosuccinimide (NBS) undergoes cyclization yielding stable bromo alkenes 87 (Scheme 28) [80,81].
Scheme 28: Cyclization of trans-acetylenic alcohol by treatment with HgCl2.
Scheme 28: Cyclization of trans-acetylenic alcohol by treatment with HgCl2.
Atta et al. reported the specific cyclization of ethynyl phenols 88 in presence of HgCl2 at ambient temperature yielding benzofuran derivatives 89. They had reported that the electron-withdrawing electronic effect at the aromatic ring promotes the cyclization reaction, whereas there is no cyclization in the absence of the withdrawing group (Scheme 29) [82].
Scheme 29: Synthesis of benzofuran derivatives in presence of HgCl2.
Scheme 29: Synthesis of benzofuran derivatives in presence of HgCl2.
Larock et al. had performed the mercuration of 4-hydroxy-2-alkyn-1-ones 90 with HgCl2 to get furylmercurials 91 via syn-addition of acetylene, which on carbonylation yielded the furan-containing carbonyl compound 92 (Scheme 30) [83]. It was proposed that initially mercuration of acetylene bonds via mercurinium like ions or π-complex takes place. Then the structure was stabilized through hydrogen bonding between alcohol and carbonyl groups (path a) or by hemiketal formation (path b). Thus, the chloride ion attacks from the frontside, producing syn-addition molecules that, upon dehydration, formed furan rings.
Scheme 30: a) Hg(II)-salt-mediated cyclization of 4-hydroxy-2-alkyn-1-ones to furan derivatives and b) its mechanistic pathway.
Scheme 30: a) Hg(II)-salt-mediated cyclization of 4-hydroxy-2-alkyn-1-ones to furan derivatives and b) its mec...
Later they had reported similar mercuration of arylacetylenes to synthesize a broad spectrum of heterocycles, namely benzofurans, benzothiophenes, isocoumarins, chromones, benzopyrans, 1,2-dihydronaphthaIenes, coumarins, and coumestan including some physiologically active heterocyclic natural products like flavones [84]. In the presence of Hg(OAc)2 in acetic acid, simple cyclization of ortho-substituted arylacetylenes 93, 95, and 97 yielded benzofurans 94, benzothiophenes 96, and indoles 98, respectively. When the carbonyl group was introduced between an aryl and a methoxy group (99) then six-membered isocoumarin ring 100 was formed, and when a carbonyl group was introduced in between an aryl and an alkyne group (101), chromone derivatives 102 were formed. On the introduction of the methylene moiety in between the aryl and methoxy group, 103 yielded isofuran derivatives 104 due to the change in regioselectivity during the cyclization. Dihydronapthalenes 106 were synthesized by cyclization of the corresponding methoxyarylalkyne derivative 105 (Scheme 31).
Scheme 31: Cyclization of arylacetylenes to synthesize carbocyclic and heterocyclic derivatives.
Scheme 31: Cyclization of arylacetylenes to synthesize carbocyclic and heterocyclic derivatives.
In 2005, Ghorai et al. reported the synthesis of a mixture of 6-membered as well as 5-membered heterocyclic compounds through a Hg(II)-salt-promoted unique cyclization–rearrangement reaction [85]. They had performed the HgCl2-promoted cyclization of O-propargyl glycolaldehyde dithioacetals 107 via their dithioketals and dithioacetals to synthesize six-membered heterocycles 108 and 109 (Scheme 32). Five-membered dihydrofuryl aldehydes were also isolated as minor products in some examples.
Scheme 32: Hg(II)-salt-promoted cyclization–rearrangement to form heterocyclic compounds.
Scheme 32: Hg(II)-salt-promoted cyclization–rearrangement to form heterocyclic compounds.
Following a similar protocol, Biswas et al. later published the HgCl2-mediated cyclization reaction of tethered alkynedithioacetals 110 to provide six- and five-membered carbocyclic and heterocyclic derivatives 111 and 112, respectively. They had observed that the formation of five-membered rings (112a–c) was preferred when substitutents were present at the alkyne terminus, whereas six-membered rings (111a,b) formed predominantly in case of unsubstituted alkyne dithioacetals (Scheme 33) [86]. They had reported the plausible mechanism for the formation of a six-membered pyranose ring follows ‘path a,' while for the formation of five-membered pyrrolidine derivatives ‘path b' was followed.
Scheme 33: a) HgCl2-mediated cyclization reaction of tethered alkyne dithioacetals; and b) proposed mechanism.
Scheme 33: a) HgCl2-mediated cyclization reaction of tethered alkyne dithioacetals; and b) proposed mechanism.
Cyclization involving allenes (>C=C=C<)
Apart from alkenes/alkynes, there are also examples where cyclization takes place involving allene functionalities. Some of the examples are discussed below.
Balasubramanian et al. reported the cyclization of aryl allenic ethers 113 on treatment with Hg(OTf)2. Compound 113 reacted with 1.1 equiv of Hg(OTf)2 at room temperature followed by reduction with alkaline NaBH4 leading to the formation of benzopyran derivatives 114 and 115. The ratio of the formation of products depends on the temperature and time of the reaction (Scheme 34) [87].
Scheme 34: Cyclization of aryl allenic ethers on treatment with Hg(OTf)2.
Scheme 34: Cyclization of aryl allenic ethers on treatment with Hg(OTf)2.
Devan et al. had developed similar types of Hg(TFA)2-mediated cyclizations of allene 116 at low temperature followed by reduction with alkaline NaBH4 to form cyclized product 117 in moderate yield [88]. The reaction was believed to proceed through Hg(II) ion-promoted electrophilic cyclization (Scheme 35).
Scheme 35: Hg(TFA)2-mediated cyclization of allene.
Scheme 35: Hg(TFA)2-mediated cyclization of allene.
Cyclization involving catalytic Hg(II) salts
Cyclization involving alkenes (>C=C<)
Apart from the stoichiometric amount used in cyclization, there is abundant literature where a catalytic amount of Hg(II) salt was employed for cyclization reactions between nucleophiles and unsaturated bonds.
The synthesis of fused polycyclic ethers by the treatment of a catalytic amount of Hg(TFA)2 with suitable starting material was demonstrated by Tan et al. [89]. They had reported a Hg(II)-catalyzed intramolecular trans-etherification reaction of 2-hydroxy-1-(γ-methoxyallyl)tetrahydropyrans 118 and 120 to the corresponding bicyclic dihydropyrans 119 and 121, respectively (Scheme 36).
Scheme 36: Hg(II)-catalyzed intramolecular trans-etherification reaction of 2-hydroxy-1-(γ-methoxyallyl)tetrahydropyran derivatives.
Scheme 36: Hg(II)-catalyzed intramolecular trans-etherification reaction of 2-hydroxy-1-(γ-methoxyallyl)tetrah...
Later, Namba et al. reported the synthesis of racemic vinylindoline derivatives 123 from N-tosylanilinoallylic alcohol derivative 122 by using 1–2 mol % of Hg(OTf)2 in CH2Cl2 at room temperature [90]. An asymmetric synthesis of vinylindoline derivatives 125 was achieved by utilizing chiral ligands like chiral binaphane (Scheme 37) [91]. They had observed that the formation of six- and seven-membered rings required elevated temperatures. Subsequently, the same group studied the cyclization of arylene 126 to furnish naphthalene derivative 127. The plausible mechanism for the formation of compound 127 proceeded consecutively with π-complex formation, Friedel–Crafts type addition, deprotonation, and finally protonation of alcohol for the elimination of water to get the final product [92].
Scheme 37: a) Cyclization of alkene derivatives by catalytic Hg(OTf)2 salts and b) mechanism of cyclization.
Scheme 37: a) Cyclization of alkene derivatives by catalytic Hg(OTf)2 salts and b) mechanism of cyclization.
A Hg(OTf)2-mediated cyclization was utilized for the synthesis of 1,4-dihydroquinoline 129 possessing a quaternary carbon center from 128 [93]. The reaction was reported via a seven-membered bicyclic hemiaminal as mentioned in the mechanism. This catalytic rearrangement protocol was successfully applied for the construction of complex carbon frameworks from various tosylanilinoallyl acetals. 4H-Chromene derivatives were also synthesized starting from phenol derivatives (Scheme 38).
Scheme 38: a) Synthesis of 1,4-dihydroquinoline derivatives by Hg(OTf)2 and b) plausible mechanism of formation.
Scheme 38: a) Synthesis of 1,4-dihydroquinoline derivatives by Hg(OTf)2 and b) plausible mechanism of formatio...
Cyclization involving alkynes (-C≡C-)
Marson et al. had developed the synthesis of substituted furans 133a–c promoted by catalytic use of Hg(II) salt through cyclization of secondary and tertiary l-alkynyl-2,3-epoxyalcohols 131a [94]. This is an example of a Hg(II)-salt-catalyzed rearrangement of 1-alkynyl-2,3-epoxy alcohols to substituted furans. The furan 133a was formed by dehydration of intermediate 132a through the corresponding oxonium cation. When R3 is an alkyl group, direct dehydration was not possible because of blocking. Later they reported a similar transformation of thiiranes to provide a wide variety of substituted thiophenes. They had synthesized substituted thiophenes 133b starting from thiiranes 131b via cyclization utilizing the catalytic amount of HgO and dil. H2SO4 at room temperature [95]. Initially, the Hg(II)-salt-catalyzed the formation of intermediate 132b which further proceeded by dehydration to yield thiophenes 133b as the final product (Scheme 39).
Scheme 39: Synthesis of Hg(II)-salt-catalyzed heteroaromatic derivatives.
Scheme 39: Synthesis of Hg(II)-salt-catalyzed heteroaromatic derivatives.
Starting with 1-alkynyl-2,3-epoxy alcohols 135, Marson et al. had reported a Hg(II)-salt-catalyzed rearrangement to produce 2,3-disubstituted-2,3-dihydropyranone derivatives 136. The stereochemistry of substituents at 2,3-positions of 2,3-dihydropyranone 136 was controlled by cis- and trans-configuration of the epoxide of starting materials (Scheme 40) [96].
Scheme 40: Hg(II)-salt-catalyzed synthesis of dihydropyranone derivatives.
Scheme 40: Hg(II)-salt-catalyzed synthesis of dihydropyranone derivatives.
Several unsaturated lactones had been synthesized from alkynoic acids via Hg(II)-salt-catalyzed cyclization reaction. For example, simple 4-pentynoic acid derivatives 137 afforded γ-methylene butyrolactones 139 in good yields via the formation of organomercural compounds 138 using catalytic mercuric oxide, mercuric acetate, or mercuric trifluoroacetate as shown in Scheme 41 [97].
Scheme 41: Hg(II)-salt-catalyzed cyclization of alkynoic acids.
Scheme 41: Hg(II)-salt-catalyzed cyclization of alkynoic acids.
Alkyne carboxylic acids also undergo oxymercuration reactions to form furan- and pyran-like derivatives. When γ-alkyne carboxylic derivative 140 was refluxed with HgO the cyclization took place to give product 141 in almost quantitative yields [98,99]. Compound 142 under refluxing conditions gave spirocyclic compound 143 as the exclusive product (Scheme 42) [99]. Propargylic triols 144 undergo Hg(OTf)2-catalyzed cyclization reaction, to produce monounsaturated spiroketal 145. Finally in the case of 1,2-dihydroxy-3-alkynes 146 comparable reaction conditions leads to the formation of 2.5-disubstitued-furan 147 rather than mono-unsaturated spiroketals [100].
Scheme 42: Hg(II)-salt-mediated cyclization of alkyne carboxylic acids and alcohol to furan, pyran, and spirocyclic derivatives.
Scheme 42: Hg(II)-salt-mediated cyclization of alkyne carboxylic acids and alcohol to furan, pyran, and spiroc...
Interestingly when 1.4-dihydroxy-5-alkyne derivatives were subjected to a Hg(OTf)2-catalyzed cyclization then oxacyclization takes place to form tetrahydropyran derivatives (Scheme 43) [101]. Later it was shown that alkynyl diol 150 when treated with 20 mol % Hg(OTf)2 followed by Et3SiH afforded bispyranoyl ketone 151, but when Hg(OTf)2 was increased (1 equiv) then fused pyran-oxocane derivative 152 was isolated [102].
Scheme 43: Hg(II)-salt-mediated cyclization of 1,4-dihydroxy-5-alkyne derivatives.
Scheme 43: Hg(II)-salt-mediated cyclization of 1,4-dihydroxy-5-alkyne derivatives.
Six-membered morpholine derivatives were also synthesized by catalytic Hg(II)-salt-induced cyclization. Yamamoto and co-workers published the intermolecular cyclization of alkynyl-carboxylic acid 153 to produce 6-membered morpholine type ring compound 154 and compound 155 [103]. The stereochemistry of the chiral amino acid was not conserved in the cyclized product hence it leads to the formation of racemic products with moderate yields (Scheme 44).
Scheme 44: Six-membered morpholine derivative formation by catalytic Hg(II)-salt-induced cyclization.
Scheme 44: Six-membered morpholine derivative formation by catalytic Hg(II)-salt-induced cyclization.
1,6-Enynes 156 underwent smooth hydroxylative carbocyclization in the presence of catalytic Hg(OTf)2. As an example, 3-methylene five-membered cyclic derivatives 157 were synthesized by Nishizawa et al. via the Hg(OTf)2-catalyzed hydroxylative carbocyclization of 1,6-enyne 156 (Scheme 45) [104]. It was observed that from 1,7-enynes, six-membered rings were formed in low to moderate yield while from 1,8-enynes, uncyclized hydrated products were isolated. as major products along with different byproducts.
Scheme 45: Hg(OTf)2-catalyzed hydroxylative carbocyclization of 1,6-enyne.
Scheme 45: Hg(OTf)2-catalyzed hydroxylative carbocyclization of 1,6-enyne.
In a Hg(OTf)2-catalyzed process, 1,6-allenynes 158 were cycloisomerized to generate allenenes 159 in moderate to good yield (Scheme 46) [105]. However, depending on the substituents, allenene and/or unexpected triene were produced as a main product for disubstituted 1,6-allenynes. It was hypothesized based on experimental evidence that alkynes would first form a π-complex with Hg(OTf)2, followed by vinylmercuration, demercuration, and eventually isomerizes to allenene.
Scheme 46: a) Hg(OTf)2-catalyzed hydroxylative carbocyclization of 1,6-enyne. b) Proposed mechanism.
Scheme 46: a) Hg(OTf)2-catalyzed hydroxylative carbocyclization of 1,6-enyne. b) Proposed mechanism.
In 2003, a carbocyclization with a catalytic quantity of mercury salt was used to efficiently synthesize dihydronapthalene derivative 161 from exemplifying benzyl derivative 160 [106]. The reported methodology was an example of the Hg(OTf)2·(TMU)3 complex promoting a moderate and efficient procedure for arylalkyne cyclization to directly afford dihydronapthalene derivatives (Scheme 47). Later, Friedel–Crafts type reaction of alkynylfuran 162 and 164 were reported in presence of the Hg(OTf)2·0.1 Sc(OTf)3 complex (5 mol %) to form six- (163) and seven-membered rings (165) in good yield. For the cyclization at 2-position of the furan the Hg(OTf)2·(TMU)3 complex was used as catalyst [107].
Scheme 47: a) Synthesis of carbocyclic derivatives using a catalytic amount of Hg(II) salt. b) Proposed mechanism for dihydronapthanene formation.
Scheme 47: a) Synthesis of carbocyclic derivatives using a catalytic amount of Hg(II) salt. b) Proposed mechan...
Later it was reported that 1-alkyn-5-ones such as 166 also undergo an effective cyclization reaction to synthesize 2-methylfuran derivatives 167 with high yield in the presence of Hg(OTf)2 as the catalyst under very mild reaction conditions (Scheme 48) [108].
Scheme 48: Cyclization of 1-alkyn-5-ones to 2-methylfuran derivatives.
Scheme 48: Cyclization of 1-alkyn-5-ones to 2-methylfuran derivatives.
Cyclization of 6-aminohex-1-yne 168 was performed by catalytic amounts of Hg(NO3)2 and generated 2-methylenepiperidine 169 initially, which further isomerizes to form 2-methyl-1,2-dehydropiperidine (170, Scheme 49) [109].
Scheme 49: Hg(NO3)2-catalyzed synthesis of 2-methylenepiperidine.
Scheme 49: Hg(NO3)2-catalyzed synthesis of 2-methylenepiperidine.
Similarly, in 2007 Kurisaki et al. had also prepared indole derivatives 172 with excellent yields from 2-ethynylaniline derivatives 171 upon the treatment of catalytic amounts of Hg(OTf)2 at room temperature. It was an example of cycloisomerization of 2-ethynylaniline derivatives utilizing mild reaction conditions (Scheme 50) [110].
Scheme 50: a) Preparation of indole derivatives through cycloisomerization of 2-ethynylaniline and b) its mechanism.
Scheme 50: a) Preparation of indole derivatives through cycloisomerization of 2-ethynylaniline and b) its mech...
Rong et al. had demonstrated the Hg(II)-salt-catalyzed enolate umpolung reaction for the efficient synthesis of various 3-indolinones and 3-coumaranones 174. They had proved that the reaction mechanism proceeds via activation of the alkynyl group with Hg(OTf)2 salt and addition of 2-chloropyridine N-oxide. The resulting activated alkynyl complex was demercurated, followed by the SN2′ reaction thus formed undergoes demercuration to yield 3-coumaranone (Scheme 51) [111].
Scheme 51: a) Hg(OTf)2-catalyzed synthesis of 3-indolinones and 3-coumaranones and b) simplified mechanism.
Scheme 51: a) Hg(OTf)2-catalyzed synthesis of 3-indolinones and 3-coumaranones and b) simplified mechanism.
Recently, a Hg(OTf)2-catalyzed one-pot cyclization of nitroalkyne 175 and alkyne had been reported to synthesize indole derivatives 176. Based on this strategy, the one-pot method to synthesize indole derivatives had been developed [112]. Similarly, benzo[c]isoxazole was also formed in excellent yields with high selectivity using this strategy. In these transformations, two Hg-carbene intermediates were proposed to be involved (Scheme 52).
Scheme 52: a) Hg(OTf)2-catalyzed one pot cyclization of nitroalkyne and b) its plausible mechanism.
Scheme 52: a) Hg(OTf)2-catalyzed one pot cyclization of nitroalkyne and b) its plausible mechanism.
Mercury-catalyzed reactions were also well known for the formation of various complex scaffolds like tricyclic pyrazinones from the corresponding starting materials. For example, Zhang et al. showed that refluxing anilide 177 in presence of a catalytic amount of Hg(OAc)2 and 90% formic acid gave the tricyclic heterocyclic scaffold 178 [113]. It involved a two-step process with the rearrangement of the primary cyclization products (Scheme 53).
Scheme 53: Synthesis of tricyclic heterocyclic scaffolds.
Scheme 53: Synthesis of tricyclic heterocyclic scaffolds.
In 2013, Lin et al. reported a Hg(II) chloride-mediated cyclization reaction of 2-alkynylphenyl alkyl sulfoxide 179 to synthesize benzothiophene derivatives 180 with good yields [114]. In this case, the reaction was believed to proceed via the initial formation of metal carbenoids followed by a sequential C–H insertion and then oxidation (Scheme 54). This methodology was later successfully utilized for the total synthesis of raloxifene and benzo[b]thiophene derivatives [115].
Scheme 54: HgCl2-mediated cyclization of 2-alkynylphenyl alkyl sulfoxide.
Scheme 54: HgCl2-mediated cyclization of 2-alkynylphenyl alkyl sulfoxide.
Cyclization involving allenes (>C=C=C<)
Hg(II) triflate salts had also been successfully employed for the arylallene 181 cyclization by Yamamoto et al [116]. The catalytic pathway was proved to involve the direct H-transfer to the vinylmercury complex from the aromatic ring. It involved Hg(OTf)2-catalyzed cyclization of aryl 1,1-disubstituted allenes with the formation of a quaternary carbon center followed by the formation of a cationic vinylmercury intermediate (Scheme 55).
Scheme 55: a) Hg(OTf)2-catalyzed cyclization of allenes and alkynes. b) Proposed mechanism of cyclization.
Scheme 55: a) Hg(OTf)2-catalyzed cyclization of allenes and alkynes. b) Proposed mechanism of cyclization.
For the synthesis of stereoselective tetrahydropyran derivatives 184, Hg(II)-catalyzed cyclization proved to be more effective than silver(I)-salt-mediated cyclization. It showed that methyl-substituted allenes undergo efficient cyclization to form polycyclic ethers under Hg(OTf)2-catalyzed conditions at lower temperature (Scheme 56) [117].
Scheme 56: Stereoselective synthesis of tetrahydropyran derivatives.
Scheme 56: Stereoselective synthesis of tetrahydropyran derivatives.
Following a similar strategy, mercury chlorate (Hg(ClO4)2) had been employed successfully as a cheap alternative to precious metals salts in the cyclization of α-allenol derivatives 185 to differently substituted 2,5-dihydrofurans 186 in an efficient and selective manner. It was also shown that from enantiopure allenyl derivatives, the desired pure cyclized product was generated by utilizing the above reaction conditions (Scheme 57) [118].
Scheme 57: a) Hg(ClO4)2-catalyzed cyclization of α-allenol derivatives. b) Simplified mechanism.
Scheme 57: a) Hg(ClO4)2-catalyzed cyclization of α-allenol derivatives. b) Simplified mechanism.
Mercury(II)-salt-mediated cyclization in total synthesis
Apart from previously described cyclization reactions, there were examples where a Hg(II)-salt-mediated cyclization had been successfully employed as one of the important steps during the total synthesis of natural products.
In 1998, a highly enantioselective total synthesis of (+)-furanomycin (190) was achieved through Hg(TFA)2-promoted cyclization of γ-hydroxyalkene derivative 187 as an intermediate stage to give a mixture of diastereomeric 2,5-disubstituted tetrahydrofurans 188a and 188b (Scheme 58) [119].
Scheme 58: Hg(TFA)2-promoted cyclization of a γ-hydroxy alkene derivative.
Scheme 58: Hg(TFA)2-promoted cyclization of a γ-hydroxy alkene derivative.
Later for the total synthesis of ventiloquinone J (194), a Hg(II)-salt-catalyzed intramolecular cyclization reaction of the ortho-allyl alcohol 192 was involved. The reaction went through the formation of a mixture of diastereoisomers 193a and 193b. After which the inseparable mixture of products underwent further oxidative demethylation and yielded the final products (Scheme 59) [120].
Scheme 59: Synthesis Hg(II)-salt-mediated cyclization of allyl alcohol for the construction of ventiloquinone J.
Scheme 59: Synthesis Hg(II)-salt-mediated cyclization of allyl alcohol for the construction of ventiloquinone ...
Kraus and co-workers had reported the synthesis of the racemic naphthohydroquinone hongconin (197) starting from the ortho-allyl alcohol derivative 195. The starting material was cyclized using a Hg(II) salts to get an inseparable mixture of products 196a and 196b in the ratio of 1:5 [121]. The synthetic route proceeded by benzylic alcohol ortho-metalation followed by a regioselective mercuri-cyclization reaction (Scheme 60).
Scheme 60: Hg(OAc)2-mediated cyclization as a key step for the synthesis of hongconin.
Scheme 60: Hg(OAc)2-mediated cyclization as a key step for the synthesis of hongconin.
During the total syntheses of (±)-fastigilin C and (–)-fastigilin C (201), 2 equiv of Hg(TFA)2 were used to synthesize key intermediate tricyclic furan compounds 199, 200, and 203. Hg(TFA)2 helped in the desired ring-formation reaction of compound 198 to afford two cyclic compounds 199 and 200 in the ratio of 6:1 with an overall 81% yield [122]. But in the case of methoxy-substituted derivative 202 only one furan derivative 203 was formed (Scheme 61).
Scheme 61: Examples of Hg(II)-salt-mediated cyclized ring formation in the syntheses of (±)-fastigilin C and (−)-fastigilin C.
Scheme 61: Examples of Hg(II)-salt-mediated cyclized ring formation in the syntheses of (±)-fastigilin C and (...
In 2007, Nishizawa et al. successfully utilized a Hg(OTf)2-induced cyclization in the key step for the synthesis of (±)-thallusin (207, Scheme 62). A complex mixture of Hg(OTf)2 and N,N-dimethylaniline (DMA) (1.2 equiv) was initially used for olefin cyclization to produce a regio- and a stereoisomeric mixture of acetate 205 after reduction and acetylation of the crude product. The Hg(OTf)2-catalyzed isomerization of the double bond in compound 205 yielded thermodynamically favorable isomer 206 as a major product [123].
Scheme 62: Formal synthesis of (±)-thallusin.
Scheme 62: Formal synthesis of (±)-thallusin.
In 2010, Ravindar et al. developed the total synthesis of the steroidal natural product hippuristanol (211) starting from 11-ketotigogenin 208 (Scheme 63). They had utilized a Hg(OTf)2-catalyzed spiroketalization reaction in their key step to form the desired ketal intermediate 210 [124]. Subsequently, in 2011, the same group reported another synthesis of hippuristanol (211) and its analog from easily available starting materials [125]. In this work, hippuristanol and some analogs were successfully synthesized utilizing a Hg(OTf)2-catalyzed cascade spiroketalization step of the 3-alkyne-1,7-diol motif. The Hg(OTf)2-catalyzed cascade spiroketalization step was proved to be more convenient than Suárez cyclization.
Scheme 63: Total synthesis of hippuristanol and its analog.
Scheme 63: Total synthesis of hippuristanol and its analog.
A Hg(TFA)2-mediated cyclization was efficiently utilized for the synthesis of highly strained tricyclo[5.2.1.01,6]decene intermediate 214 containing a cyclobutane ring (Scheme 64). Compound 213 is an important precursor for the asymmetric total synthesis of solanoeclepin A. The formation of β-hydroxyketone 213 was achieved by Hg(TFA)2-mediated cyclization of compound 212 using TFA/H2O (1.7: 1) as the solvent. In presence of other mercury compounds like HgO and Hg(OAc)2 no product or starting material was recovered [126].
Scheme 64: Total synthesis of solanoeclepin A.
Scheme 64: Total synthesis of solanoeclepin A.
Spiro-skeleton structures are found in many natural products and synthesizing stereoselective spiro-skeletons has always been difficult for organic chemists. Morimoto and co-workers were the first to disclose the Hg(OTf)2-catalyzed cycloisomerization of amino ynone to produce the azaspiro skeleton. Later, this methodology was successfully used for the synthesis of several spiroskeleton structures. Natural products such as histrionicotoxin alkaloids 218 (Scheme 65) [127,128] and lepadoformine [129,130] were being successfully synthesized using this methodology for spirocyclic ring structure synthesis. The proposed mechanism proceeded initially with aminoketal formation by 6-exo-dig intramolecular oxymercuration, followed by Petasis–Ferrier-type cyclization, and finally nucleophilic addition of mercuric enolate to iminium results in the formation of azaspiro structure.
Scheme 65: a) Synthesis of Hg(OTf)2-catalyzed azaspiro structure for the formation of natural products. b) Proposed mechanism for azaspiro formation.
Scheme 65: a) Synthesis of Hg(OTf)2-catalyzed azaspiro structure for the formation of natural products. b) Pro...
Conclusion
In conclusion, this review summarizes Hg(II)-salt-mediated cyclization reactions either for direct synthesis of cyclized products or as a part of the total synthesis of important natural products. Different Hg(II) salts were used stoichiometrically or catalytically depending upon the nature of functional groups and reactants. However, the reactivity of different unsaturated bonds involved in the cyclization primarily depends on the nucleophile as well as nature of functional groups attached to unsaturated bonds. When alkenes are linked to activating groups like methoxy or hydroxy, a catalytic quantity of Hg(II) ions is required for cyclization. Nothing can be predicted in case of alkynes; however, the presence of a strong nucleophile promotes the Hg(II)-salt-catalyzed cyclization of allenes in most circumstances. In cyclization reactions, Hg(OTf)2 showed to be the most effective and versatile of all Hg(II) salts. Mercury(II) salts can also be used to cyclize unsaturated bonds in a regio- and diastereoselective manner. Apart from toxicity concerns, Hg(II) salts are cheap, stable, and versatile in terms of reactivity, making them a viable option to similar transition metal catalysts.
References
-
Lautens, M.; Klute, W.; Tam, W. Chem. Rev. 1996, 96, 49–92. doi:10.1021/cr950016l
Return to citation in text: [1] -
Zhou, F.; Li, C.-J. Chem. Sci. 2019, 10, 34–46. doi:10.1039/c8sc04271c
Return to citation in text: [1] -
Lou, J.; Wang, Q.; Wu, P.; Wang, H.; Zhou, Y.-G.; Yu, Z. Chem. Soc. Rev. 2020, 49, 4307–4359. doi:10.1039/c9cs00837c
Return to citation in text: [1] -
Beller, M.; Bolm, C., Eds. Transition Metals for Organic Synthesis: Building Blocks and Fine Chemicals, 1st ed.; Wiley-VCH: Weinheim, Germany, 2004. doi:10.1002/9783527619405
Return to citation in text: [1] -
Sharma, R.; Kour, P.; Kumar, A. J. Chem. Sci. 2018, 130, 73. doi:10.1007/s12039-018-1466-8
Return to citation in text: [1] -
Tanaka, K., Ed. Transition-Metal-Mediated Aromatic Ring Construction; John Wiley & Sons: Hoboken, NJ, USA, 2013. doi:10.1002/9781118629871
Return to citation in text: [1] -
Solé, D.; Fernández, I., Eds. Advances in Transition-Metal Mediated Heterocyclic Synthesis; Academic Press: London, UK, 2018.
Return to citation in text: [1] -
Biffis, A.; Centomo, P.; Del Zotto, A.; Zecca, M. Chem. Rev. 2018, 118, 2249–2295. doi:10.1021/acs.chemrev.7b00443
Return to citation in text: [1] -
Saranya, S.; Rohit, K. R.; Radhika, S.; Anilkumar, G. Org. Biomol. Chem. 2019, 17, 8048–8061. doi:10.1039/c9ob01538h
Return to citation in text: [1] -
Ruiz-Castillo, P.; Buchwald, S. L. Chem. Rev. 2016, 116, 12564–12649. doi:10.1021/acs.chemrev.6b00512
Return to citation in text: [1] -
Duarah, G.; Kaishap, P. P.; Begum, T.; Gogoi, S. Adv. Synth. Catal. 2019, 361, 654–672. doi:10.1002/adsc.201800755
Return to citation in text: [1] -
Seretis, A.; Diamantopoulou, P.; Thanou, I.; Tzevelekidis, P.; Fakas, C.; Lilas, P.; Papadogianakis, G. Front. Chem. (Lausanne, Switz.) 2020, 8, 221. doi:10.3389/fchem.2020.00221
Return to citation in text: [1] -
Johansson, J. R.; Beke-Somfai, T.; Said Stålsmeden, A.; Kann, N. Chem. Rev. 2016, 116, 14726–14768. doi:10.1021/acs.chemrev.6b00466
Return to citation in text: [1] -
Fan, X.; Liu, C.-H.; Yu, Z.-X. Rhodium(I)-Catalyzed Cycloadditions Involving Vinylcyclopropanes and Their Derivatives. In Rhodium Catalysis in Organic Synthesis; Tanaka, K., Ed.; Wiley-VCH: Weinheim, Germany, 2019; pp 229–276. doi:10.1002/9783527811908.ch10
Return to citation in text: [1] -
Wee, A. G. H. Curr. Org. Synth. 2006, 3, 499–555. doi:10.2174/157017906778699512
Return to citation in text: [1] -
Evans, P. A., Ed. Modern Rhodium‐Catalyzed Organic Reactions, 1st ed.; Wiley-VCH: Weinheim, Germany, 2005. doi:10.1002/3527604693
Return to citation in text: [1] -
Mondal, M.; Bora, U. RSC Adv. 2013, 3, 18716. doi:10.1039/c3ra42480d
Return to citation in text: [1] -
Carney, J. R.; Dillon, B. R.; Thomas, S. P. Eur. J. Org. Chem. 2016, 3912–3929. doi:10.1002/ejoc.201600018
Return to citation in text: [1] -
Concellón, J. M.; Rodríguez-Solla, H.; del Amo, V. Chem. – Eur. J. 2008, 14, 10184–10191. doi:10.1002/chem.200800796
Return to citation in text: [1] -
Hashmi, A. S. K. Chem. Rev. 2007, 107, 3180–3211. doi:10.1021/cr000436x
Return to citation in text: [1] -
Campeau, D.; León Rayo, D. F.; Mansour, A.; Muratov, K.; Gagosz, F. Chem. Rev. 2021, 121, 8756–8867. doi:10.1021/acs.chemrev.0c00788
Return to citation in text: [1] -
Boorman, T. C.; Larrosa, I. Chem. Soc. Rev. 2011, 40, 1910–1925. doi:10.1039/c0cs00098a
Return to citation in text: [1] -
Abbiati, G.; Rossi, E. Beilstein J. Org. Chem. 2014, 10, 481–513. doi:10.3762/bjoc.10.46
Return to citation in text: [1] -
Wu, Y.-C.; Xiao, Y.-T.; Yang, Y.-Z.; Song, R.-J.; Li, J.-H. ChemCatChem 2020, 12, 5312–5329. doi:10.1002/cctc.202000900
Return to citation in text: [1] -
Sekine, K.; Yamada, T. Chem. Soc. Rev. 2016, 45, 4524–4532. doi:10.1039/c5cs00895f
Return to citation in text: [1] -
Larock, R. C. Mercury. Comprehensive Organometallic Chemistry II; Elsevier: Amsterdam, Netherlands, 1995; pp 389–435. doi:10.1016/b978-008046519-7.00100-3
Return to citation in text: [1] -
Vogt, R. R.; Nieuwland, J. A. J. Am. Chem. Soc. 1921, 43, 2071–2081. doi:10.1021/ja01442a010
Return to citation in text: [1] -
Ramalingan, C.; Park, Y.-T. J. Org. Chem. 2007, 72, 4536–4538. doi:10.1021/jo070297k
Return to citation in text: [1] -
Gong, Y.; Cao, Z.-Y.; Shi, Y.-B.; Zhou, F.; Zhou, Y.; Zhou, J. Org. Chem. Front. 2019, 6, 3989–3995. doi:10.1039/c9qo01049a
Return to citation in text: [1] -
Masuhara, T. Nihon Shika Ishikai Zasshi 1975, 28, 128.
Return to citation in text: [1] -
Clarkson, T. W.; Magos, L. Crit. Rev. Toxicol. 2006, 36, 609–662. doi:10.1080/10408440600845619
Return to citation in text: [1] -
Leyva-Pérez, A.; Corma, A. Angew. Chem., Int. Ed. 2012, 51, 614–635. doi:10.1002/anie.201101726
Return to citation in text: [1] -
Larock, R. C. Angew. Chem., Int. Ed. Engl. 1978, 17, 27–37. doi:10.1002/anie.197800271
Return to citation in text: [1] -
Lattes, A. Chem. Heterocycl. Compd. 1975, 11, 4–17. doi:10.1007/bf00945261
Return to citation in text: [1] -
Kaur, N. Synth. Commun. 2018, 48, 2715–2749. doi:10.1080/00397911.2018.1497657
Return to citation in text: [1] -
Sand, J. Ber. Dtsch. Chem. Ges. 1901, 34, 1385–1394. doi:10.1002/cber.19010340207
Return to citation in text: [1] -
Biilmann, E. Ber. Dtsch. Chem. Ges. 1900, 33, 1641–1655. doi:10.1002/cber.19000330235
Return to citation in text: [1] -
Summerbell, R. K.; Stephens, J. R. J. Am. Chem. Soc. 1954, 76, 6401–6407. doi:10.1021/ja01653a046
Return to citation in text: [1] -
Summerbell, R. K.; Stephens, J. R. J. Am. Chem. Soc. 1954, 76, 731–734. doi:10.1021/ja01632a028
Return to citation in text: [1] -
Summerbell, R. K.; Lestina, G.; Waite, H. J. Am. Chem. Soc. 1957, 79, 234–237. doi:10.1021/ja01558a061
Return to citation in text: [1] -
Pougny, J.-R.; Nassr, M. A. M.; Sinaÿ, P. J. Chem. Soc., Chem. Commun. 1981, 375–376. doi:10.1039/c39810000375
Return to citation in text: [1] -
Boschetti, A.; Nicotra, F.; Panza, L.; Russo, G. J. Org. Chem. 1988, 53, 4181–4185. doi:10.1021/jo00253a005
Return to citation in text: [1] -
Nolen, E. G.; Kurish, A. J.; Potter, J. M.; Donahue, L. A.; Orlando, M. D. Org. Lett. 2005, 7, 3383–3386. doi:10.1021/ol051341q
Return to citation in text: [1] -
Nicotra, F.; Panza, L.; Ronchetti, F.; Toma, L. Tetrahedron Lett. 1984, 25, 5937–5938. doi:10.1016/s0040-4039(01)81725-1
Return to citation in text: [1] -
Reitz, A. B.; Nortey, S. O.; Maryanoff, B. E. Tetrahedron Lett. 1985, 26, 3915–3918. doi:10.1016/s0040-4039(00)98686-6
Return to citation in text: [1] -
Andrey, O.; Glanzmann, C.; Landais, Y.; Parra-Rapado, L. Tetrahedron 1997, 53, 2835–2854. doi:10.1016/s0040-4020(97)00003-3
Return to citation in text: [1] [2] -
Pulido, F. J.; Barbero, A.; Val, P.; Diez, A.; González-Ortega, A. Eur. J. Org. Chem. 2012, 5350–5356. doi:10.1002/ejoc.201200666
Return to citation in text: [1] [2] [3] -
Mohapatra, D. K.; Mohapatra, S.; Gurjar, M. K. Tetrahedron Lett. 2006, 47, 5943–5947. doi:10.1016/j.tetlet.2006.06.049
Return to citation in text: [1] -
Enderlin, G.; Nielsen, P. J. Org. Chem. 2008, 73, 6891–6894. doi:10.1021/jo801081t
Return to citation in text: [1] -
Perie, J. J.; Laval, J. P.; Roussel, J.; Lattes, A. Tetrahedron 1972, 28, 675–699. doi:10.1016/0040-4020(72)84031-6
Return to citation in text: [1] -
Roussel, J.; Perie, J. J.; Laval, J. P.; Lattes, A. Tetrahedron 1972, 28, 701–716. doi:10.1016/0040-4020(72)84032-8
Return to citation in text: [1] -
Tokuda, M.; Yamada, Y.; Takagi, T.; Suginome, H.; Furusaki, A. Tetrahedron 1987, 43, 281–296. doi:10.1016/s0040-4020(01)89956-7
Return to citation in text: [1] -
Roubaud, V.; Moigne, F. L.; Mercier, A.; Tordo, P. Phosphorus, Sulfur Silicon Relat. Elem. 1994, 86, 39–54. doi:10.1080/10426509408018386
Return to citation in text: [1] [2] -
Le Moigne, F.; Mercier, A.; Tordo, P. Tetrahedron Lett. 1991, 32, 3841–3844. doi:10.1016/s0040-4039(00)79391-9
Return to citation in text: [1] -
Stipa, P.; Finet, J. P.; Le Moigne, F.; Tordo, P. J. Org. Chem. 1993, 58, 4465–4468. doi:10.1021/jo00068a049
Return to citation in text: [1] -
Dembkowski, L.; Finet, J. P.; Fréjaville, C.; Le Moigne, F.; Maurin, R.; Mercier, A.; Pages, P.; Stipa, P.; Tordo, P. Free Radical Res. Commun. 1993, 19 (Suppl. 1), s23–s32. doi:10.3109/10715769309056s23
Return to citation in text: [1] -
Le Moigne, F.; Tordo, P. J. Org. Chem. 1994, 59, 3365–3367. doi:10.1021/jo00091a024
Return to citation in text: [1] -
Roubaud, V.; Moigne, F. L.; Mercier, A.; Tordo, P. Synth. Commun. 1996, 26, 1507–1516. doi:10.1080/00397919608003517
Return to citation in text: [1] -
Barluenga, J.; Pérez-Prieto, J.; Bayón, A. M.; Asensio, G. Tetrahedron 1984, 40, 1199–1204. doi:10.1016/s0040-4020(01)99327-5
Return to citation in text: [1] -
Barluenga, J.; Perez-Prieto, J.; Asensio, G.; Garcia-Granda, S.; Salvado, M. A. Tetrahedron 1992, 48, 3813–3826. doi:10.1016/s0040-4020(01)92272-0
Return to citation in text: [1] -
Carroll, F. I.; Hu, X.; Navarro, H. A.; Deschamps, J.; Abdrakhmanova, G. R.; Damaj, M. I.; Martin, B. R. J. Med. Chem. 2006, 49, 3244–3250. doi:10.1021/jm060122n
Return to citation in text: [1] -
Peçanha, E. P.; Verli, H.; Rodrigues, C. R.; Barreiro, E. J.; Fraga, C. A. M. Tetrahedron Lett. 2002, 43, 1607–1611. doi:10.1016/s0040-4039(02)00074-6
Return to citation in text: [1] -
Nixon, J. R.; Cudd, M. A.; Porter, N. A. J. Org. Chem. 1978, 43, 4048–4052. doi:10.1021/jo00415a014
Return to citation in text: [1] -
Bloodworth, A. J.; Tallant, N. A. J. Chem. Soc., Chem. Commun. 1992, 428–429. doi:10.1039/c39920000428
Return to citation in text: [1] -
Kurbanov, M.; Semenovsky, A. V.; Smit, W. A.; Shmelev, L. V.; Kucherov, V. F. Tetrahedron Lett. 1972, 13, 2175–2178. doi:10.1016/s0040-4039(01)84798-5
Return to citation in text: [1] -
Hoye, T. R.; Caruso, A. J.; Kurth, M. J. J. Org. Chem. 1981, 46, 3550–3552. doi:10.1021/jo00330a037
Return to citation in text: [1] -
Amoroso, R.; Cardillo, G.; Tomasini, C. Tetrahedron Lett. 1990, 31, 6413–6416. doi:10.1016/s0040-4039(00)97079-5
Return to citation in text: [1] -
Cardillo, G.; Amoroso, R.; Cardillo, G.; Tomasini, C. Heterocycles 1992, 34, 349. doi:10.3987/com-91-5930
Return to citation in text: [1] -
Harding, K. E.; Stephens, R.; Hollingsworth, D. R. Tetrahedron Lett. 1984, 25, 4631–4632. doi:10.1016/s0040-4039(01)91218-3
Return to citation in text: [1] -
Harding, K. E.; Hollingsworth, D. R. Tetrahedron Lett. 1988, 29, 3789–3792. doi:10.1016/s0040-4039(00)82115-2
Return to citation in text: [1] -
Takacs, J. M.; Helle, M. A.; Yang, L. Tetrahedron Lett. 1989, 30, 1777–1780. doi:10.1016/s0040-4039(00)99577-7
Return to citation in text: [1] -
Takacs, J. M.; Helle, M. A.; Takusagawa, F. Tetrahedron Lett. 1989, 30, 7321–7324. doi:10.1016/s0040-4039(00)70687-3
Return to citation in text: [1] -
Majumdar, K. C.; Thyagarajan, B. S. J. Heterocycl. Chem. 1972, 9, 489–494. doi:10.1002/jhet.5570090305
Return to citation in text: [1] -
Bates, D. K.; Jones, M. C. J. Org. Chem. 1978, 43, 3856–3861. doi:10.1021/jo00414a015
Return to citation in text: [1] -
Bates, D. K.; Jones, M. C. J. Org. Chem. 1978, 43, 3775–3776. doi:10.1021/jo00413a032
Return to citation in text: [1] -
Thyagarajan, B. S.; Majumdar, K. C.; Bates, D. K. J. Heterocycl. Chem. 1975, 12, 59–66. doi:10.1002/jhet.5570120110
Return to citation in text: [1] -
Balasubramanian, K. K.; Virupaksha Reddy, K.; Nagarajan, R. Tetrahedron Lett. 1973, 14, 5003–5004. doi:10.1016/s0040-4039(01)87633-4
Return to citation in text: [1] -
Drouin, J.; Boaventura, M. A.; Conia, J. M. J. Am. Chem. Soc. 1985, 107, 1726–1729. doi:10.1021/ja00292a045
Return to citation in text: [1] -
Riediker, M.; Schwartz, J. J. Am. Chem. Soc. 1982, 104, 5842–5844. doi:10.1021/ja00385a074
Return to citation in text: [1] [2] -
Compernolle, F.; Mao, H.; Tahri, A.; Kozlecki, T.; Van der Eycken, E.; Medaer, B.; Hoornaert, G. J. Tetrahedron Lett. 2002, 43, 3011–3015. doi:10.1016/s0040-4039(02)00404-5
Return to citation in text: [1] -
Mao, H.; Koukni, M.; Kozlecki, T.; Compernolle, F.; Hoornaert, G. J. Tetrahedron Lett. 2002, 43, 8697–8700. doi:10.1016/s0040-4039(02)02111-1
Return to citation in text: [1] -
Atta, A. K.; Kim, S.-B.; Heo, J.; Cho, D.-G. Org. Lett. 2013, 15, 1072–1075. doi:10.1021/ol4000873
Return to citation in text: [1] -
Larock, R. C.; Liu, C. L. J. Org. Chem. 1983, 48, 2151–2158. doi:10.1021/jo00161a007
Return to citation in text: [1] -
Larock, R. C.; Harrison, L. W. J. Am. Chem. Soc. 1984, 106, 4218–4227. doi:10.1021/ja00327a026
Return to citation in text: [1] -
Ghorai, S.; Bhattacharjya, A. Org. Lett. 2005, 7, 207–210. doi:10.1021/ol047893a
Return to citation in text: [1] -
Biswas, G.; Ghorai, S.; Bhattacharjya, A. Org. Lett. 2006, 8, 313–316. doi:10.1021/ol0527274
Return to citation in text: [1] -
Balasubramanian, T.; Balasubramanian, K. K. Tetrahedron Lett. 1991, 32, 6641–6644. doi:10.1016/0040-4039(91)80243-y
Return to citation in text: [1] -
Devan, B.; Rajagopalan, K. Synth. Commun. 1994, 24, 1691–1700. doi:10.1080/00397919408010171
Return to citation in text: [1] -
Tan, D. S.; Schreiber, S. L. Tetrahedron Lett. 2000, 41, 9509–9513. doi:10.1016/s0040-4039(00)01658-0
Return to citation in text: [1] -
Namba, K.; Nakagawa, Y.; Yamamoto, H.; Imagawa, H.; Nishizawa, M. Synlett 2008, 1719–1723. doi:10.1055/s-2008-1077881
Return to citation in text: [1] -
Yamamoto, H.; Ho, E.; Namba, K.; Imagawa, H.; Nishizawa, M. Chem. – Eur. J. 2010, 16, 11271–11274. doi:10.1002/chem.201001656
Return to citation in text: [1] -
Namba, K.; Yamamoto, H.; Sasaki, I.; Mori, K.; Imagawa, H.; Nishizawa, M. Org. Lett. 2008, 10, 1767–1770. doi:10.1021/ol800450x
Return to citation in text: [1] -
Namba, K.; Kanaki, M.; Suto, H.; Nishizawa, M.; Tanino, K. Org. Lett. 2012, 14, 1222–1225. doi:10.1021/ol2034492
Return to citation in text: [1] -
Marson, C. M.; Harper, S.; Wrigglesworth, R. J. Chem. Soc., Chem. Commun. 1994, 1879. doi:10.1039/c39940001879
Return to citation in text: [1] -
Marson, C. M.; Campbell, J. Tetrahedron Lett. 1997, 38, 7785–7788. doi:10.1016/s0040-4039(97)01819-4
Return to citation in text: [1] -
Marson, C. M.; Harper, S.; Oare, C. A.; Walsgrove, T. J. Org. Chem. 1998, 63, 3798–3799. doi:10.1021/jo9715837
Return to citation in text: [1] -
Krafft, G. A.; Katzenellenbogen, J. A. J. Am. Chem. Soc. 1981, 103, 5459–5466. doi:10.1021/ja00408a030
Return to citation in text: [1] -
Yamamoto, M. J. Chem. Soc., Chem. Commun. 1978, 649–650. doi:10.1039/c39780000649
Return to citation in text: [1] -
Yamamoto, M.; Yoshitake, M.; Yamada, K. J. Chem. Soc., Chem. Commun. 1983, 991–992. doi:10.1039/c39830000991
Return to citation in text: [1] [2] -
Ravindar, K.; Sridhar Reddy, M.; Deslongchamps, P. Org. Lett. 2011, 13, 3178–3181. doi:10.1021/ol201102x
Return to citation in text: [1] -
McDonald, F. E.; Ishida, K.; Hurtak, J. A. Tetrahedron 2013, 69, 7746–7758. doi:10.1016/j.tet.2013.04.138
Return to citation in text: [1] -
Hurtak, J.; McDonald, F. Synlett 2017, 28, 2951–2955. doi:10.1055/s-0036-1588562
Return to citation in text: [1] -
Yamamoto, M.; Tanaka, S.; Naruchi, K.; Yamada, K. Synthesis 1982, 850–852. doi:10.1055/s-1982-29970
Return to citation in text: [1] -
Nishizawa, M.; Yadav, V. K.; Skwarczynski, M.; Takao, H.; Imagawa, H.; Sugihara, T. Org. Lett. 2003, 5, 1609–1611. doi:10.1021/ol034201u
Return to citation in text: [1] -
Sim, S. H.; Lee, S. I.; Seo, J.; Chung, Y. K. J. Org. Chem. 2007, 72, 9818–9821. doi:10.1021/jo701591e
Return to citation in text: [1] -
Nishizawa, M.; Takao, H.; Yadav, V. K.; Imagawa, H.; Sugihara, T. Org. Lett. 2003, 5, 4563–4565. doi:10.1021/ol035622e
Return to citation in text: [1] -
Yamamoto, H.; Sasaki, I.; Imagawa, H.; Nishizawa, M. Org. Lett. 2007, 9, 1399–1402. doi:10.1021/ol070335m
Return to citation in text: [1] -
Imagawa, H.; Kurisaki, T.; Nishizawa, M. Org. Lett. 2004, 6, 3679–3681. doi:10.1021/ol048730p
Return to citation in text: [1] -
Müller, T. E.; Pleier, A.-K. J. Chem. Soc., Dalton Trans. 1999, 583. doi:10.1039/a808938h
Return to citation in text: [1] -
Kurisaki, T.; Naniwa, T.; Yamamoto, H.; Imagawa, H.; Nishizawa, M. Tetrahedron Lett. 2007, 48, 1871–1874. doi:10.1016/j.tetlet.2006.12.120
Return to citation in text: [1] -
Rong, Z.; Hu, W.; Dai, N.; Qian, G. Org. Lett. 2020, 22, 3286–3290. doi:10.1021/acs.orglett.0c01096
Return to citation in text: [1] -
Zheng, M.; Chen, K.; Zhu, S. Synthesis 2017, 49, 4173–4182. doi:10.1055/s-0036-1588416
Return to citation in text: [1] -
Zhang, J.; Haider, N. ARKIVOC 2016, No. iii, 125–133. doi:10.3998/ark.5550190.p009.495
Return to citation in text: [1] -
Lin, C.-H.; Chen, C.-C.; Wu, M.-J. Chem. – Eur. J. 2013, 19, 2578–2581. doi:10.1002/chem.201202704
Return to citation in text: [1] -
Wen, S.-M.; Lin, C.-H.; Chen, C.-C.; Wu, M.-J. Tetrahedron 2018, 74, 2493–2499. doi:10.1016/j.tet.2018.03.067
Return to citation in text: [1] -
Yamamoto, H.; Ueda, M.; Yamasaki, N.; Fujii, A.; Sasaki, I.; Igawa, K.; Kasai, Y.; Imagawa, H.; Nishizawa, M. Org. Lett. 2016, 18, 2864–2867. doi:10.1021/acs.orglett.6b01144
Return to citation in text: [1] -
Suzuki, Y.; Kuwabara, A.; Koizumi, Y.; Mori, Y. Tetrahedron 2013, 69, 9086–9095. doi:10.1016/j.tet.2013.08.026
Return to citation in text: [1] -
Alcaide, B.; Almendros, P.; Luna, A.; Soriano, E. J. Org. Chem. 2015, 80, 7050–7057. doi:10.1021/acs.joc.5b00887
Return to citation in text: [1] -
Kang, S. H.; Lee, S. B. Chem. Commun. 1998, 761–762. doi:10.1039/a800727f
Return to citation in text: [1] -
Giles, R. G. F.; Green, I. R.; van Eeden, N. Synthesis 2004, 1601–1608. doi:10.1055/s-2004-822370
Return to citation in text: [1] -
Kraus, G. A.; Li, J.; Gordon, M.; Jensen, J. H. J. Org. Chem. 1994, 59, 2219–2222. doi:10.1021/jo00087a044
Return to citation in text: [1] -
Tanis, S. P.; Robinson, E. D.; McMills, M. C.; Watt, W. J. Am. Chem. Soc. 1992, 114, 8349–8362. doi:10.1021/ja00048a003
Return to citation in text: [1] -
Nishizawa, M.; Iyenaga, T.; Kurisaki, T.; Yamamoto, H.; Sharfuddin, M.; Namba, K.; Imagawa, H.; Shizuri, Y.; Matsuo, Y. Tetrahedron Lett. 2007, 48, 4229–4233. doi:10.1016/j.tetlet.2007.04.075
Return to citation in text: [1] -
Ravindar, K.; Reddy, M. S.; Lindqvist, L.; Pelletier, J.; Deslongchamps, P. Org. Lett. 2010, 12, 4420–4423. doi:10.1021/ol1019663
Return to citation in text: [1] -
Ravindar, K.; Reddy, M. S.; Lindqvist, L.; Pelletier, J.; Deslongchamps, P. J. Org. Chem. 2011, 76, 1269–1284. doi:10.1021/jo102054r
Return to citation in text: [1] -
Chuang, H.-Y.; Isobe, M. Org. Lett. 2014, 16, 4166–4169. doi:10.1021/ol501858w
Return to citation in text: [1] -
Matsumura, K.; Nishikawa, K.; Yoshida, H.; Doe, M.; Morimoto, Y. RSC Adv. 2018, 8, 11296–11303. doi:10.1039/c8ra02011f
Return to citation in text: [1] -
Matsumura, K.; Nishikawa, K.; Yoshida, H.; Niwa, T.; Fushii, Y.; Doe, M.; Morimoto, Y. Chem. – Asian J. 2021, 16, 1882–1886. doi:10.1002/asia.202100383
Return to citation in text: [1] -
Nishikawa, K.; Yamauchi, K.; Kikuchi, S.; Ezaki, S.; Koyama, T.; Nokubo, H.; Matsumura, K.; Kodama, T.; Kumagai, M.; Morimoto, Y. Chem. – Eur. J. 2017, 23, 9535–9545. doi:10.1002/chem.201701475
Return to citation in text: [1] -
Nishikawa, K.; Kikuchi, S.; Ezaki, S.; Koyama, T.; Nokubo, H.; Kodama, T.; Tachi, Y.; Morimoto, Y. Org. Lett. 2015, 17, 5772–5775. doi:10.1021/acs.orglett.5b02867
Return to citation in text: [1]
98. | Yamamoto, M. J. Chem. Soc., Chem. Commun. 1978, 649–650. doi:10.1039/c39780000649 |
99. | Yamamoto, M.; Yoshitake, M.; Yamada, K. J. Chem. Soc., Chem. Commun. 1983, 991–992. doi:10.1039/c39830000991 |
99. | Yamamoto, M.; Yoshitake, M.; Yamada, K. J. Chem. Soc., Chem. Commun. 1983, 991–992. doi:10.1039/c39830000991 |
96. | Marson, C. M.; Harper, S.; Oare, C. A.; Walsgrove, T. J. Org. Chem. 1998, 63, 3798–3799. doi:10.1021/jo9715837 |
97. | Krafft, G. A.; Katzenellenbogen, J. A. J. Am. Chem. Soc. 1981, 103, 5459–5466. doi:10.1021/ja00408a030 |
95. | Marson, C. M.; Campbell, J. Tetrahedron Lett. 1997, 38, 7785–7788. doi:10.1016/s0040-4039(97)01819-4 |
104. | Nishizawa, M.; Yadav, V. K.; Skwarczynski, M.; Takao, H.; Imagawa, H.; Sugihara, T. Org. Lett. 2003, 5, 1609–1611. doi:10.1021/ol034201u |
102. | Hurtak, J.; McDonald, F. Synlett 2017, 28, 2951–2955. doi:10.1055/s-0036-1588562 |
103. | Yamamoto, M.; Tanaka, S.; Naruchi, K.; Yamada, K. Synthesis 1982, 850–852. doi:10.1055/s-1982-29970 |
100. | Ravindar, K.; Sridhar Reddy, M.; Deslongchamps, P. Org. Lett. 2011, 13, 3178–3181. doi:10.1021/ol201102x |
101. | McDonald, F. E.; Ishida, K.; Hurtak, J. A. Tetrahedron 2013, 69, 7746–7758. doi:10.1016/j.tet.2013.04.138 |
109. | Müller, T. E.; Pleier, A.-K. J. Chem. Soc., Dalton Trans. 1999, 583. doi:10.1039/a808938h |
110. | Kurisaki, T.; Naniwa, T.; Yamamoto, H.; Imagawa, H.; Nishizawa, M. Tetrahedron Lett. 2007, 48, 1871–1874. doi:10.1016/j.tetlet.2006.12.120 |
107. | Yamamoto, H.; Sasaki, I.; Imagawa, H.; Nishizawa, M. Org. Lett. 2007, 9, 1399–1402. doi:10.1021/ol070335m |
108. | Imagawa, H.; Kurisaki, T.; Nishizawa, M. Org. Lett. 2004, 6, 3679–3681. doi:10.1021/ol048730p |
105. | Sim, S. H.; Lee, S. I.; Seo, J.; Chung, Y. K. J. Org. Chem. 2007, 72, 9818–9821. doi:10.1021/jo701591e |
127. | Matsumura, K.; Nishikawa, K.; Yoshida, H.; Doe, M.; Morimoto, Y. RSC Adv. 2018, 8, 11296–11303. doi:10.1039/c8ra02011f |
128. | Matsumura, K.; Nishikawa, K.; Yoshida, H.; Niwa, T.; Fushii, Y.; Doe, M.; Morimoto, Y. Chem. – Asian J. 2021, 16, 1882–1886. doi:10.1002/asia.202100383 |
106. | Nishizawa, M.; Takao, H.; Yadav, V. K.; Imagawa, H.; Sugihara, T. Org. Lett. 2003, 5, 4563–4565. doi:10.1021/ol035622e |
126. | Chuang, H.-Y.; Isobe, M. Org. Lett. 2014, 16, 4166–4169. doi:10.1021/ol501858w |
129. | Nishikawa, K.; Yamauchi, K.; Kikuchi, S.; Ezaki, S.; Koyama, T.; Nokubo, H.; Matsumura, K.; Kodama, T.; Kumagai, M.; Morimoto, Y. Chem. – Eur. J. 2017, 23, 9535–9545. doi:10.1002/chem.201701475 |
130. | Nishikawa, K.; Kikuchi, S.; Ezaki, S.; Koyama, T.; Nokubo, H.; Kodama, T.; Tachi, Y.; Morimoto, Y. Org. Lett. 2015, 17, 5772–5775. doi:10.1021/acs.orglett.5b02867 |
125. | Ravindar, K.; Reddy, M. S.; Lindqvist, L.; Pelletier, J.; Deslongchamps, P. J. Org. Chem. 2011, 76, 1269–1284. doi:10.1021/jo102054r |
113. | Zhang, J.; Haider, N. ARKIVOC 2016, No. iii, 125–133. doi:10.3998/ark.5550190.p009.495 |
114. | Lin, C.-H.; Chen, C.-C.; Wu, M.-J. Chem. – Eur. J. 2013, 19, 2578–2581. doi:10.1002/chem.201202704 |
111. | Rong, Z.; Hu, W.; Dai, N.; Qian, G. Org. Lett. 2020, 22, 3286–3290. doi:10.1021/acs.orglett.0c01096 |
112. | Zheng, M.; Chen, K.; Zhu, S. Synthesis 2017, 49, 4173–4182. doi:10.1055/s-0036-1588416 |
76. | Thyagarajan, B. S.; Majumdar, K. C.; Bates, D. K. J. Heterocycl. Chem. 1975, 12, 59–66. doi:10.1002/jhet.5570120110 |
77. | Balasubramanian, K. K.; Virupaksha Reddy, K.; Nagarajan, R. Tetrahedron Lett. 1973, 14, 5003–5004. doi:10.1016/s0040-4039(01)87633-4 |
73. | Majumdar, K. C.; Thyagarajan, B. S. J. Heterocycl. Chem. 1972, 9, 489–494. doi:10.1002/jhet.5570090305 |
74. | Bates, D. K.; Jones, M. C. J. Org. Chem. 1978, 43, 3856–3861. doi:10.1021/jo00414a015 |
75. | Bates, D. K.; Jones, M. C. J. Org. Chem. 1978, 43, 3775–3776. doi:10.1021/jo00413a032 |
1. | Lautens, M.; Klute, W.; Tam, W. Chem. Rev. 1996, 96, 49–92. doi:10.1021/cr950016l |
2. | Zhou, F.; Li, C.-J. Chem. Sci. 2019, 10, 34–46. doi:10.1039/c8sc04271c |
3. | Lou, J.; Wang, Q.; Wu, P.; Wang, H.; Zhou, Y.-G.; Yu, Z. Chem. Soc. Rev. 2020, 49, 4307–4359. doi:10.1039/c9cs00837c |
4. | Beller, M.; Bolm, C., Eds. Transition Metals for Organic Synthesis: Building Blocks and Fine Chemicals, 1st ed.; Wiley-VCH: Weinheim, Germany, 2004. doi:10.1002/9783527619405 |
14. | Fan, X.; Liu, C.-H.; Yu, Z.-X. Rhodium(I)-Catalyzed Cycloadditions Involving Vinylcyclopropanes and Their Derivatives. In Rhodium Catalysis in Organic Synthesis; Tanaka, K., Ed.; Wiley-VCH: Weinheim, Germany, 2019; pp 229–276. doi:10.1002/9783527811908.ch10 |
15. | Wee, A. G. H. Curr. Org. Synth. 2006, 3, 499–555. doi:10.2174/157017906778699512 |
16. | Evans, P. A., Ed. Modern Rhodium‐Catalyzed Organic Reactions, 1st ed.; Wiley-VCH: Weinheim, Germany, 2005. doi:10.1002/3527604693 |
84. | Larock, R. C.; Harrison, L. W. J. Am. Chem. Soc. 1984, 106, 4218–4227. doi:10.1021/ja00327a026 |
11. | Duarah, G.; Kaishap, P. P.; Begum, T.; Gogoi, S. Adv. Synth. Catal. 2019, 361, 654–672. doi:10.1002/adsc.201800755 |
12. | Seretis, A.; Diamantopoulou, P.; Thanou, I.; Tzevelekidis, P.; Fakas, C.; Lilas, P.; Papadogianakis, G. Front. Chem. (Lausanne, Switz.) 2020, 8, 221. doi:10.3389/fchem.2020.00221 |
13. | Johansson, J. R.; Beke-Somfai, T.; Said Stålsmeden, A.; Kann, N. Chem. Rev. 2016, 116, 14726–14768. doi:10.1021/acs.chemrev.6b00466 |
8. | Biffis, A.; Centomo, P.; Del Zotto, A.; Zecca, M. Chem. Rev. 2018, 118, 2249–2295. doi:10.1021/acs.chemrev.7b00443 |
9. | Saranya, S.; Rohit, K. R.; Radhika, S.; Anilkumar, G. Org. Biomol. Chem. 2019, 17, 8048–8061. doi:10.1039/c9ob01538h |
10. | Ruiz-Castillo, P.; Buchwald, S. L. Chem. Rev. 2016, 116, 12564–12649. doi:10.1021/acs.chemrev.6b00512 |
82. | Atta, A. K.; Kim, S.-B.; Heo, J.; Cho, D.-G. Org. Lett. 2013, 15, 1072–1075. doi:10.1021/ol4000873 |
5. | Sharma, R.; Kour, P.; Kumar, A. J. Chem. Sci. 2018, 130, 73. doi:10.1007/s12039-018-1466-8 |
6. | Tanaka, K., Ed. Transition-Metal-Mediated Aromatic Ring Construction; John Wiley & Sons: Hoboken, NJ, USA, 2013. doi:10.1002/9781118629871 |
7. | Solé, D.; Fernández, I., Eds. Advances in Transition-Metal Mediated Heterocyclic Synthesis; Academic Press: London, UK, 2018. |
83. | Larock, R. C.; Liu, C. L. J. Org. Chem. 1983, 48, 2151–2158. doi:10.1021/jo00161a007 |
26. | Larock, R. C. Mercury. Comprehensive Organometallic Chemistry II; Elsevier: Amsterdam, Netherlands, 1995; pp 389–435. doi:10.1016/b978-008046519-7.00100-3 |
27. | Vogt, R. R.; Nieuwland, J. A. J. Am. Chem. Soc. 1921, 43, 2071–2081. doi:10.1021/ja01442a010 |
28. | Ramalingan, C.; Park, Y.-T. J. Org. Chem. 2007, 72, 4536–4538. doi:10.1021/jo070297k |
29. | Gong, Y.; Cao, Z.-Y.; Shi, Y.-B.; Zhou, F.; Zhou, Y.; Zhou, J. Org. Chem. Front. 2019, 6, 3989–3995. doi:10.1039/c9qo01049a |
79. | Riediker, M.; Schwartz, J. J. Am. Chem. Soc. 1982, 104, 5842–5844. doi:10.1021/ja00385a074 |
23. | Abbiati, G.; Rossi, E. Beilstein J. Org. Chem. 2014, 10, 481–513. doi:10.3762/bjoc.10.46 |
24. | Wu, Y.-C.; Xiao, Y.-T.; Yang, Y.-Z.; Song, R.-J.; Li, J.-H. ChemCatChem 2020, 12, 5312–5329. doi:10.1002/cctc.202000900 |
25. | Sekine, K.; Yamada, T. Chem. Soc. Rev. 2016, 45, 4524–4532. doi:10.1039/c5cs00895f |
80. | Compernolle, F.; Mao, H.; Tahri, A.; Kozlecki, T.; Van der Eycken, E.; Medaer, B.; Hoornaert, G. J. Tetrahedron Lett. 2002, 43, 3011–3015. doi:10.1016/s0040-4039(02)00404-5 |
81. | Mao, H.; Koukni, M.; Kozlecki, T.; Compernolle, F.; Hoornaert, G. J. Tetrahedron Lett. 2002, 43, 8697–8700. doi:10.1016/s0040-4039(02)02111-1 |
20. | Hashmi, A. S. K. Chem. Rev. 2007, 107, 3180–3211. doi:10.1021/cr000436x |
21. | Campeau, D.; León Rayo, D. F.; Mansour, A.; Muratov, K.; Gagosz, F. Chem. Rev. 2021, 121, 8756–8867. doi:10.1021/acs.chemrev.0c00788 |
22. | Boorman, T. C.; Larrosa, I. Chem. Soc. Rev. 2011, 40, 1910–1925. doi:10.1039/c0cs00098a |
78. | Drouin, J.; Boaventura, M. A.; Conia, J. M. J. Am. Chem. Soc. 1985, 107, 1726–1729. doi:10.1021/ja00292a045 |
17. | Mondal, M.; Bora, U. RSC Adv. 2013, 3, 18716. doi:10.1039/c3ra42480d |
18. | Carney, J. R.; Dillon, B. R.; Thomas, S. P. Eur. J. Org. Chem. 2016, 3912–3929. doi:10.1002/ejoc.201600018 |
19. | Concellón, J. M.; Rodríguez-Solla, H.; del Amo, V. Chem. – Eur. J. 2008, 14, 10184–10191. doi:10.1002/chem.200800796 |
79. | Riediker, M.; Schwartz, J. J. Am. Chem. Soc. 1982, 104, 5842–5844. doi:10.1021/ja00385a074 |
87. | Balasubramanian, T.; Balasubramanian, K. K. Tetrahedron Lett. 1991, 32, 6641–6644. doi:10.1016/0040-4039(91)80243-y |
88. | Devan, B.; Rajagopalan, K. Synth. Commun. 1994, 24, 1691–1700. doi:10.1080/00397919408010171 |
85. | Ghorai, S.; Bhattacharjya, A. Org. Lett. 2005, 7, 207–210. doi:10.1021/ol047893a |
86. | Biswas, G.; Ghorai, S.; Bhattacharjya, A. Org. Lett. 2006, 8, 313–316. doi:10.1021/ol0527274 |
93. | Namba, K.; Kanaki, M.; Suto, H.; Nishizawa, M.; Tanino, K. Org. Lett. 2012, 14, 1222–1225. doi:10.1021/ol2034492 |
94. | Marson, C. M.; Harper, S.; Wrigglesworth, R. J. Chem. Soc., Chem. Commun. 1994, 1879. doi:10.1039/c39940001879 |
91. | Yamamoto, H.; Ho, E.; Namba, K.; Imagawa, H.; Nishizawa, M. Chem. – Eur. J. 2010, 16, 11271–11274. doi:10.1002/chem.201001656 |
92. | Namba, K.; Yamamoto, H.; Sasaki, I.; Mori, K.; Imagawa, H.; Nishizawa, M. Org. Lett. 2008, 10, 1767–1770. doi:10.1021/ol800450x |
89. | Tan, D. S.; Schreiber, S. L. Tetrahedron Lett. 2000, 41, 9509–9513. doi:10.1016/s0040-4039(00)01658-0 |
90. | Namba, K.; Nakagawa, Y.; Yamamoto, H.; Imagawa, H.; Nishizawa, M. Synlett 2008, 1719–1723. doi:10.1055/s-2008-1077881 |
47. | Pulido, F. J.; Barbero, A.; Val, P.; Diez, A.; González-Ortega, A. Eur. J. Org. Chem. 2012, 5350–5356. doi:10.1002/ejoc.201200666 |
46. | Andrey, O.; Glanzmann, C.; Landais, Y.; Parra-Rapado, L. Tetrahedron 1997, 53, 2835–2854. doi:10.1016/s0040-4020(97)00003-3 |
49. | Enderlin, G.; Nielsen, P. J. Org. Chem. 2008, 73, 6891–6894. doi:10.1021/jo801081t |
59. | Barluenga, J.; Pérez-Prieto, J.; Bayón, A. M.; Asensio, G. Tetrahedron 1984, 40, 1199–1204. doi:10.1016/s0040-4020(01)99327-5 |
60. | Barluenga, J.; Perez-Prieto, J.; Asensio, G.; Garcia-Granda, S.; Salvado, M. A. Tetrahedron 1992, 48, 3813–3826. doi:10.1016/s0040-4020(01)92272-0 |
61. | Carroll, F. I.; Hu, X.; Navarro, H. A.; Deschamps, J.; Abdrakhmanova, G. R.; Damaj, M. I.; Martin, B. R. J. Med. Chem. 2006, 49, 3244–3250. doi:10.1021/jm060122n |
54. | Le Moigne, F.; Mercier, A.; Tordo, P. Tetrahedron Lett. 1991, 32, 3841–3844. doi:10.1016/s0040-4039(00)79391-9 |
55. | Stipa, P.; Finet, J. P.; Le Moigne, F.; Tordo, P. J. Org. Chem. 1993, 58, 4465–4468. doi:10.1021/jo00068a049 |
56. | Dembkowski, L.; Finet, J. P.; Fréjaville, C.; Le Moigne, F.; Maurin, R.; Mercier, A.; Pages, P.; Stipa, P.; Tordo, P. Free Radical Res. Commun. 1993, 19 (Suppl. 1), s23–s32. doi:10.3109/10715769309056s23 |
57. | Le Moigne, F.; Tordo, P. J. Org. Chem. 1994, 59, 3365–3367. doi:10.1021/jo00091a024 |
53. | Roubaud, V.; Moigne, F. L.; Mercier, A.; Tordo, P. Phosphorus, Sulfur Silicon Relat. Elem. 1994, 86, 39–54. doi:10.1080/10426509408018386 |
58. | Roubaud, V.; Moigne, F. L.; Mercier, A.; Tordo, P. Synth. Commun. 1996, 26, 1507–1516. doi:10.1080/00397919608003517 |
52. | Tokuda, M.; Yamada, Y.; Takagi, T.; Suginome, H.; Furusaki, A. Tetrahedron 1987, 43, 281–296. doi:10.1016/s0040-4020(01)89956-7 |
53. | Roubaud, V.; Moigne, F. L.; Mercier, A.; Tordo, P. Phosphorus, Sulfur Silicon Relat. Elem. 1994, 86, 39–54. doi:10.1080/10426509408018386 |
50. | Perie, J. J.; Laval, J. P.; Roussel, J.; Lattes, A. Tetrahedron 1972, 28, 675–699. doi:10.1016/0040-4020(72)84031-6 |
51. | Roussel, J.; Perie, J. J.; Laval, J. P.; Lattes, A. Tetrahedron 1972, 28, 701–716. doi:10.1016/0040-4020(72)84032-8 |
62. | Peçanha, E. P.; Verli, H.; Rodrigues, C. R.; Barreiro, E. J.; Fraga, C. A. M. Tetrahedron Lett. 2002, 43, 1607–1611. doi:10.1016/s0040-4039(02)00074-6 |
63. | Nixon, J. R.; Cudd, M. A.; Porter, N. A. J. Org. Chem. 1978, 43, 4048–4052. doi:10.1021/jo00415a014 |
64. | Bloodworth, A. J.; Tallant, N. A. J. Chem. Soc., Chem. Commun. 1992, 428–429. doi:10.1039/c39920000428 |
71. | Takacs, J. M.; Helle, M. A.; Yang, L. Tetrahedron Lett. 1989, 30, 1777–1780. doi:10.1016/s0040-4039(00)99577-7 |
72. | Takacs, J. M.; Helle, M. A.; Takusagawa, F. Tetrahedron Lett. 1989, 30, 7321–7324. doi:10.1016/s0040-4039(00)70687-3 |
69. | Harding, K. E.; Stephens, R.; Hollingsworth, D. R. Tetrahedron Lett. 1984, 25, 4631–4632. doi:10.1016/s0040-4039(01)91218-3 |
70. | Harding, K. E.; Hollingsworth, D. R. Tetrahedron Lett. 1988, 29, 3789–3792. doi:10.1016/s0040-4039(00)82115-2 |
67. | Amoroso, R.; Cardillo, G.; Tomasini, C. Tetrahedron Lett. 1990, 31, 6413–6416. doi:10.1016/s0040-4039(00)97079-5 |
68. | Cardillo, G.; Amoroso, R.; Cardillo, G.; Tomasini, C. Heterocycles 1992, 34, 349. doi:10.3987/com-91-5930 |
65. | Kurbanov, M.; Semenovsky, A. V.; Smit, W. A.; Shmelev, L. V.; Kucherov, V. F. Tetrahedron Lett. 1972, 13, 2175–2178. doi:10.1016/s0040-4039(01)84798-5 |
66. | Hoye, T. R.; Caruso, A. J.; Kurth, M. J. J. Org. Chem. 1981, 46, 3550–3552. doi:10.1021/jo00330a037 |
120. | Giles, R. G. F.; Green, I. R.; van Eeden, N. Synthesis 2004, 1601–1608. doi:10.1055/s-2004-822370 |
121. | Kraus, G. A.; Li, J.; Gordon, M.; Jensen, J. H. J. Org. Chem. 1994, 59, 2219–2222. doi:10.1021/jo00087a044 |
118. | Alcaide, B.; Almendros, P.; Luna, A.; Soriano, E. J. Org. Chem. 2015, 80, 7050–7057. doi:10.1021/acs.joc.5b00887 |
116. | Yamamoto, H.; Ueda, M.; Yamasaki, N.; Fujii, A.; Sasaki, I.; Igawa, K.; Kasai, Y.; Imagawa, H.; Nishizawa, M. Org. Lett. 2016, 18, 2864–2867. doi:10.1021/acs.orglett.6b01144 |
117. | Suzuki, Y.; Kuwabara, A.; Koizumi, Y.; Mori, Y. Tetrahedron 2013, 69, 9086–9095. doi:10.1016/j.tet.2013.08.026 |
115. | Wen, S.-M.; Lin, C.-H.; Chen, C.-C.; Wu, M.-J. Tetrahedron 2018, 74, 2493–2499. doi:10.1016/j.tet.2018.03.067 |
36. | Sand, J. Ber. Dtsch. Chem. Ges. 1901, 34, 1385–1394. doi:10.1002/cber.19010340207 |
37. | Biilmann, E. Ber. Dtsch. Chem. Ges. 1900, 33, 1641–1655. doi:10.1002/cber.19000330235 |
38. | Summerbell, R. K.; Stephens, J. R. J. Am. Chem. Soc. 1954, 76, 6401–6407. doi:10.1021/ja01653a046 |
35. | Kaur, N. Synth. Commun. 2018, 48, 2715–2749. doi:10.1080/00397911.2018.1497657 |
32. | Leyva-Pérez, A.; Corma, A. Angew. Chem., Int. Ed. 2012, 51, 614–635. doi:10.1002/anie.201101726 |
124. | Ravindar, K.; Reddy, M. S.; Lindqvist, L.; Pelletier, J.; Deslongchamps, P. Org. Lett. 2010, 12, 4420–4423. doi:10.1021/ol1019663 |
33. | Larock, R. C. Angew. Chem., Int. Ed. Engl. 1978, 17, 27–37. doi:10.1002/anie.197800271 |
122. | Tanis, S. P.; Robinson, E. D.; McMills, M. C.; Watt, W. J. Am. Chem. Soc. 1992, 114, 8349–8362. doi:10.1021/ja00048a003 |
30. | Masuhara, T. Nihon Shika Ishikai Zasshi 1975, 28, 128. |
31. | Clarkson, T. W.; Magos, L. Crit. Rev. Toxicol. 2006, 36, 609–662. doi:10.1080/10408440600845619 |
123. | Nishizawa, M.; Iyenaga, T.; Kurisaki, T.; Yamamoto, H.; Sharfuddin, M.; Namba, K.; Imagawa, H.; Shizuri, Y.; Matsuo, Y. Tetrahedron Lett. 2007, 48, 4229–4233. doi:10.1016/j.tetlet.2007.04.075 |
41. | Pougny, J.-R.; Nassr, M. A. M.; Sinaÿ, P. J. Chem. Soc., Chem. Commun. 1981, 375–376. doi:10.1039/c39810000375 |
39. | Summerbell, R. K.; Stephens, J. R. J. Am. Chem. Soc. 1954, 76, 731–734. doi:10.1021/ja01632a028 |
40. | Summerbell, R. K.; Lestina, G.; Waite, H. J. Am. Chem. Soc. 1957, 79, 234–237. doi:10.1021/ja01558a061 |
48. | Mohapatra, D. K.; Mohapatra, S.; Gurjar, M. K. Tetrahedron Lett. 2006, 47, 5943–5947. doi:10.1016/j.tetlet.2006.06.049 |
47. | Pulido, F. J.; Barbero, A.; Val, P.; Diez, A.; González-Ortega, A. Eur. J. Org. Chem. 2012, 5350–5356. doi:10.1002/ejoc.201200666 |
46. | Andrey, O.; Glanzmann, C.; Landais, Y.; Parra-Rapado, L. Tetrahedron 1997, 53, 2835–2854. doi:10.1016/s0040-4020(97)00003-3 |
47. | Pulido, F. J.; Barbero, A.; Val, P.; Diez, A.; González-Ortega, A. Eur. J. Org. Chem. 2012, 5350–5356. doi:10.1002/ejoc.201200666 |
44. | Nicotra, F.; Panza, L.; Ronchetti, F.; Toma, L. Tetrahedron Lett. 1984, 25, 5937–5938. doi:10.1016/s0040-4039(01)81725-1 |
45. | Reitz, A. B.; Nortey, S. O.; Maryanoff, B. E. Tetrahedron Lett. 1985, 26, 3915–3918. doi:10.1016/s0040-4039(00)98686-6 |
42. | Boschetti, A.; Nicotra, F.; Panza, L.; Russo, G. J. Org. Chem. 1988, 53, 4181–4185. doi:10.1021/jo00253a005 |
43. | Nolen, E. G.; Kurish, A. J.; Potter, J. M.; Donahue, L. A.; Orlando, M. D. Org. Lett. 2005, 7, 3383–3386. doi:10.1021/ol051341q |
© 2021 Mandal et al.; licensee Beilstein-Institut.
This is an Open Access article under the terms of the Creative Commons Attribution License (https://creativecommons.org/licenses/by/4.0). Please note that the reuse, redistribution and reproduction in particular requires that the author(s) and source are credited and that individual graphics may be subject to special legal provisions.
The license is subject to the Beilstein Journal of Organic Chemistry terms and conditions: (https://www.beilstein-journals.org/bjoc/terms)